Novel medicines development for cancer treatment
Posted: 24 June 2010 |
The pharmaceutical industry has reached a critical phase in its evolution. The cost and time to develop novel medicines has become unsustainable3. Reasons for this may include a much higher demand on evidence of safety and efficacy, rapidly increasing costs of contract research and the tremendous pressure on pricing and reimbursement from regulators and payers expecting higher benefit rates than the current average for newly launched products. Concomitant with these pressures we observe an ever decreasing R&D productivity which acerbates the problem2.
In this context, it is important to remind ourselves of the task to be achieved. First, a suitable target has to be identified whose inhibition will result in major effects on tumour growth and survival.
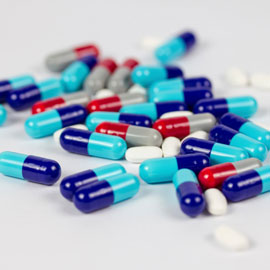
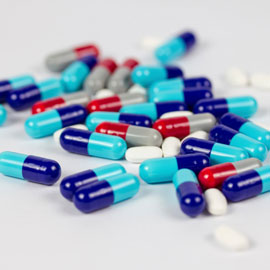
The pharmaceutical industry has reached a critical phase in its evolution. The cost and time to develop novel medicines has become unsustainable3. Reasons for this may include a much higher demand on evidence of safety and efficacy, rapidly increasing costs of contract research and the tremendous pressure on pricing and reimbursement from regulators and payers expecting higher benefit rates than the current average for newly launched products. Concomitant with these pressures we observe an ever decreasing R&D productivity which acerbates the problem2.
In this context, it is important to remind ourselves of the task to be achieved. First, a suitable target has to be identified whose inhibition will result in major effects on tumour growth and survival. In oncology, targets are traditionally derived from our knowledge of the cell cycle, cell proliferation and apoptosis. This approach leads to a wealth of reagents that potently inhibit tumour growth in vitro and in animal models. However, in humans, anti-tumour effects are much more limited. Thus, pharmaceutical researchers are focusing more and more on tumour biology as a starting point, trying to understand the molecular principles driving the growth, survival and metastasis of tumours and tumour sub-types. Identifying the driving molecules not only provides novel targets but being able to analyse the precise molecular difference between tumour and normal tissue allows identifying the correct patient population for treatment.
The second challenge is to find a balance between potency, safety and exposure that is sufficient to achieve anti-tumour effects. This is not a trivial undertaking as any attempts to improve one of these aspects through chemical modification most often has a negative effect on the other parameters. Furthermore, the effect assessment has to be carried out in animals, severely limiting the number of possible chemical variants that can be assessed.
The limited power of preclinical animal models poses an additional critical problem. While cell line xenograft models are adequate for optimising the activity balance of compounds as mentioned above they are of very limited use for predicting the sensitivity of particular tumour (sub)-types. It is therefore increasingly important to apply more predictive models such as fresh human tumours in mice or 3D organotypic cell culture7.
To address the issue of benefit rate and improved evidence for safety and efficacy, many companies have installed biomarker efforts to support clinical decision making. This review focuses on three types of biomarkers: pre-clinical and clinical safety biomarkers, pharmacodynamic (PD) biomarkers and predictive biomarkers.
Safety biomarkers have been used for quite some time and serve to identify safety concerns preclincially to prevent unexpected severe adverse effects (SAEs) in clinical assessment of novel compounds. These usually include hERG and other cardiovascular safety tests as well as standard tests for organ function, mainly liver and kidney.
Regarding the early assessment of efficacy, it is seen as critical to provide evidence for positive target modulation in phase I clinical trials. Robust evidence for pharmacodynamic activity in the absence of clinical responses of a compound in Phase I is often required to transition into Phase II. This is of particular importance because, due to the late stage and heterogeneity of phase I patient populations, clinical responses are not to be expected. Furthermore, the establishment of a biologically effective dose (BED) based on PD measurements is critical in cases where a maximal tolerated dose (MTD) cannot be found (e.g. with biological compounds). Especially knowing the extent and duration of target inhibition (or less frequently target activation) is necessary to achieve effects on tumour growth and survival is key. This is usually achieved by preclinical pharmacokinetic/ pharmacodynamic (PK/PD) modelling. Such models are obtained by measuring plasma exposure, tumour size and PD endpoints for target activity (e.g. the level of P-kinase or P-substrate for kinase targets) over time and at various doses4. Moreover, it is important to assess some fundamental questions about the target, the compound and their interaction in a human body. These considerations have been termed the ‘Pharmacological Audit Trail’ by Collins and Workman1. Answers to very basic questions should be found before major investments in Phase II development are warranted. First, these issues are explored in preclinical animal models and then later, verification is sought in the clinic.
These questions include:
- Is the target expressed in the treated tumour? This is obviously a critical question since there is no expected effect in the absence of the target
- Is the target dys-regulated in any tumour types or subtypes of tumours, for example, are there known activating mutations (like EGFGR in non small cell lung cancer) or is the gene amplified (like HER2 in a subset of breast cancers)?
Knowing the frequency and number of tumour types where the target is dys-regulated can be a strong lead for a predictive marker. An assay detecting such target aberrations can be used to identify tumour (sub)-types and patients likely to receive superior clinical benefit.
Are there genetic variants of the target that show increased or decreased sensitivity to the compound (such the hypersensitive L858R and the resistant T790M mutations in EGFR)? The sensitivity of different known genetic variants can be tested preclincially in cell lines or potentially even on isolated target protein. Knowing the frequency of hypersensitive and resistant variants is needed to inform the development plan to focus on sensitive popu – lations and avoid resistant ones. The latter may not be trivial as resistant variants seem to spring up upon treatment. This is a cruel reminder of Darwinian principles where pre-existing minor variants come to be the dominant form based on evolutionary pressure; survival of the fittest tumour cells, so to speak. It is, however, important to be able to detect resistant variants as soon as they emerge to consider alternative treatment options as early as possible.
Is there evidence for target modulation to an extent and for long enough to expect an effect on tumour growth as predicted by pre – clinical PD modelling? This assessment relies on several crucial components:
- The expectation as informed by the pre clinical PK/PD model
- Robust assays – Immunochemistry (IHC), ELISA or RT-PCR
- High quality tissue samples, both tumour and surrogate tissue
Serial samples are required for PD assessment. Thus, strong hypotheses must be available to warrant rather invasive tumour biopsies.
In this regard, the design of Phase I trials is also of critical importance. Traditional Phase I trials have used standard dose escalation schemes based on a modified Fibonacci design, also known as the Korn 3+3 design6 with the primary goal to reach a MTD as quickly as possible and focus clinical assessments mainly on PK and safety. This maximal tolerated dose would also become the recommended dose for phase II trials. More recently, with the advent of targeted therapies, it has become necessary to find different designs accommodating for the possibility that a MTD cannot be reached.
In this case, one would assess PD effects in surrogate tissues such as skin, hair follicle, plasma, peripheral blood mononuclear cells etc. at lower dose levels to establish the expected PK/PD relationship. Exploring the PD effect as a function of exposure is expected to yield a biologically effective dose (BED) that can be related back to preclinical PK/PD models and allows predicting the extent and duration of a PD response required for anti-tumour efficacy. Once biologically meaningful dose levels, based on PD results, are reached, one would require mandatory, serial tumour biopsies to assess the PK/PD relationship in tumours. This allows establishing either an MTD – and the associated PD effect – or a maximal BED which would become the recommended Phase II dose. At the end of such a trial, the investigators should have a clear understanding of the probability that the treatment could result in meaningful clinical activity in the appropriate target population.
In some cases, clinical activity is observed at doses that turn out not to be tolerable long term and thus alternative dosing regimens (other than the usual daily administration) have to be explored. In such a case, the PD effect results obtained during dose escalation are invaluable to assess whether a meaningful and potentially clinically active exposure has been achieved using an alternative administration schedule. Even in the absence of clear clinical responses, a positive decision can be made regarding progression to Phase II if PD effects are observed that are comparable in extent and duration to those observed in the presence of clinical activity at continuous dosing.
Perhaps the biggest hurdle for novel targeted therapies is finding those patient populations that receive substantial clinical benefit as opposed to barely statistically significant improvements over existing standards of care. Being able to identify such patients using diagnostic assays is key to personalised medicine and is likely to become a requirement for payers to reimburse for expensive treatment courses. Often, predictive markers are derived from knowledge about target dys-regulation, for example, mutations and gene amplification9. It is, however, not always clear what marker assay or com – bination of marker assays results in the best performance for patient selection during clinical development and in medical practice.
For example, in NSCLC, both mutations and amplification plays a role. The population with the highest overall response rate (retrospectively) are patients whose tumours carry the Δ-exon-19 deletion of the EGFR gene which results in a constitutively active EGF receptor8. However, this mutation is only present in approximately three per cent of an overall Caucasian population and it would be very difficult to conduct clinical trials where only three out of 100 screened patients are actually treated.
Another sub-population however, those testing positive for both EGFR gene amplification and EGFR protein over-expression by IHC, show an overall response rate of about 41 per cent8 and represent approximately 23 per cent of the overall population. Thus, this combination of markers could form the basis of a viable selection strategy. Since these insights have been gleaned from retrospective meta-analyses, it is likely to not be so easy to arrive at the same conclusions in the absence of this wealth of clinical experience. Nevertheless, careful con – sideration of potential assays and their combination is critical for formulating a success-ful predictive biomarker strategy with a view of developing companion diagnostics for patient selection. In the case of EGFR inhibitors, using such a patient selection strategy could reduce the number of patients required for a phase III pivotal trial by three to four-fold and substantially reduce the time and cost.
In summary, the development of novel medicines is a highly multidisciplinary, long term and expensive undertaking. The current poor success rate can be improved by modifying early clinical development to include several compounds per target and by applying pristine PK/PD analyses in Phase I thus reducing the number of programs failing in Phase II and beyond through more evidence based decision making. Predictive markers derived from target knowledge and ex vivo pharmacology can reduce the time and cost of clinical development by focusing on populations with an optimal likelihood of clinical benefit. Furthermore, companion diagnostics derived from predictive biomarkers can improve the success rate in the effort for regulatory and payer acceptance.
References
- Collins, I. & Workman, P (2006) New approaches to molecular cancer therapeutics. Nature Chemical Biology 2: 689-700
- Hughes, B. (2009) 2008 FDA drug approvals. Nature Reviews Drug Discovery 8: 93-96
- Paul, S.M., et al. (2010) How to improve R&D productivity: the pharmaceutical industry’s grand challenge. Nature Review Drug Discovery 9: 203-214
- Yamazaki S. et al (2008) Pharmacokinetic-Pharmacodynamic Modeling of Biomarker Response and Tumor Growth Inhibition to an Orally Available cMet Kinase Inhibitor in Human Tumor Xenograft Mouse Models. Drug Metabolism Disposition 36:1267–1274
- Hunsberger, S. et al. (2005) Dose escalation trial designs based on a molecularly targeted endpoint. Statistics in Medicine 24: 2171–2181
- Korn E.L. & Simon, R. (1993) Using the tolerable dose diagram in the design of phase I combination chemotherapy trials. Journal of Clinical Oncology 11:794-801
- Hall, B., (2009) A new age in drug development: biomarkers and the tumour microenvironment. European Pharmaceutical Review, 1: 38-41
- Hirsch F.R et al., (2007) Combination of EGFR gene copy number and protein expression predicts outcome for advanced non-small-cell lung cancer patients treated with gefitinib. Annals of Oncology 18: 752-760
- Schilsky R. L., (2010) Personalized medicine in oncology: the future is now. Nature Reviews Drug Discovery 9: 363-366
About the Author
Hans (Johann) Winkler is Senior Director at Ortho Biotech Oncology Research & Development, leading Oncology Biomarker Programs globally. Hans originally joined the J&JPRD Drug Discovery team in Beerse in 2003 as the Head of Functional Genomics, a newly created department combining genomic technologies and bioinformatics. Before joining J&JPRD, he was Director and Global Program Manager for Target Validation Technologies at AstraZeneca, with responsibility for target validation technology assessment and acquisition across all disease areas globally. He held positions of increasing responsibility at Zeneca, including team leader in Target Discovery and Senior Group Leader and Associate Director responsible for TI/TV activities and technology assessment and acquisition across several disease areas. Prior to this, he was a Scientist and Instructor at Harvard Medical School’s Deaconess Hospital (now Beth Israel Deaconess Hospital) in Boston, where he worked on endothelial cell biology and immunobiology in a xenotransplantation context, after work as a Scientist and Group Leader at the Vienna International Research Cooperation Center in Vienna (Novartis Research Institute) working on gene regulation in endothelial cells. Hans studied Biochemistry at the University of Vienna after which he was awarded a Fulbright fellowship to attend graduate school in the U.S. where he obtained a Master’s degree in Molecular Biology (working on Caenorrhabditis elegans) from the University of Houston in 1986. Hans then returned to Vienna to work on his PhD thesis. He completed his postdoctoral work at the Institute for Molecular Pathology. Hans is co-author of some forty original, peer-reviewed publications and book chapters and inventor on two patents. Contact the author: [email protected]