What are the challenges in developing and delivering lipid nanoparticle mRNA-based vaccines?
Posted: 29 June 2021 | Andrew Bright (CPI), Stuart Jamieson (CPI Biologics) | No comments yet
In response to the COVID-19 pandemic caused by the SARS-CoV-2 virus, the emergence of RNA vaccine technology has enabled rapid development of critical vaccines. Traditional vaccine production methods makerapid development challenging, requiring cell line production of viral proteins and laborious purification development, which requires extensive effort and long timelines. RNA vaccine technology utilises lipid nanoparticle-encapsulated mRNA to deliver targeted genetic information to patient cells, allowing them to produce the viral protein, triggering an immune response. In this article Andrew Bright and Stuart Jamieson highlight and discuss the unique challenges of this breakthrough vaccine technology through development and delivery.
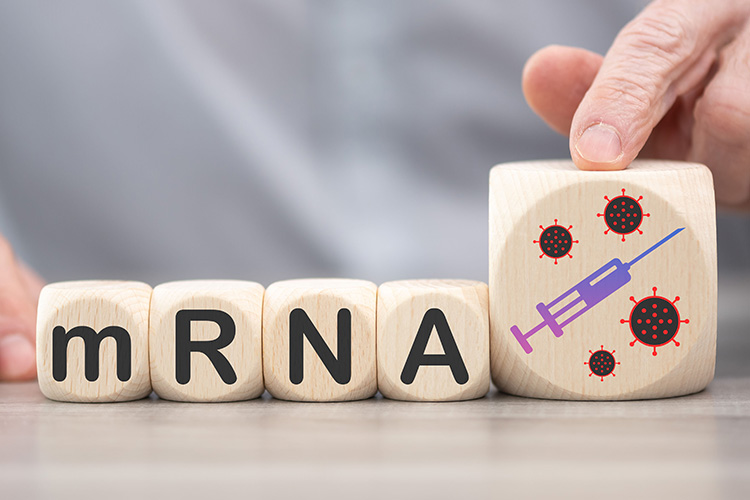
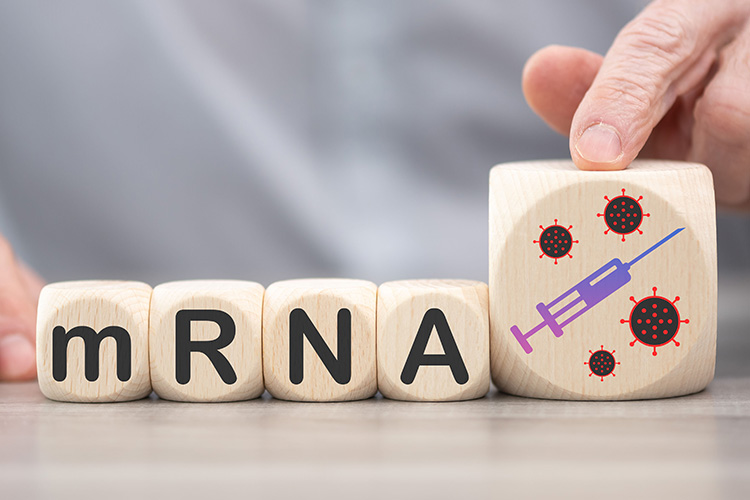
THE SARS-CoV-2 virus and the pandemic caused by it has led to an unprecedented and accelerated effort to develop a vaccine. By 3 March 2021, 177 vaccine candidates for the prevention of COVID-19 were in development.1 Prior to 2020, the development of a new vaccine was a long process taking an average of 10 years – the fastest being the mumps vaccine that was developed in four years in the 1960s. The most advanced SARS-CoV-2 vaccines, however, are based on the use of mRNA lipid nanoparticle technology, with the Moderna (mRNA‑1273) and Pfizer-BioNTech (BNT162b2) vaccines being approved for use in under 10 months while still meeting the stringent regulatory standards for safety and effectiveness.2
In these new generation vaccines, mRNA encodes the genetic information to produce a target antigen, such as the spike protein that is found on the surface of the SARS-CoV-2 virus. This mRNA is then encapsulated in lipid nanoparticles for injection.3 Once injected into a person, the mRNA is taken up into the cells and their own intracellular translation machinery is triggered to produce the antigen protein. These produced antigens then trigger the body’s immune response and the production of antibodies, which ultimately protect the body against future infections from that virus.
The potential of mRNA vaccines was first demonstrated in the 1990s4,5 but did not lead to a substantial investment in developing mRNA therapeutics, primarily due to its short half-life and inefficient in vivo delivery.6 However, the transient nature of mRNA is a benefit where time-controlled expression of a protein is required, as is the case in a vaccination. In addition, due to the small dose requirements, independence from biological systems for production (cell line construction and development) and the potential for platform process development, mRNA vaccines can be faster, cheaper, more adaptable and easier to mass produce than tradition vaccines. Although mRNA lipid nanoparticle vaccines have now been approved and other companies are continuing to develop this technology, there are several challenges to overcome in the development and delivery of the new vaccines based on this technology, including:
- Identification of the target antigen
- mRNA synthesis and purification
- mRNA Encapsulation
- Formulation, stability and analytical characterisation of the mRNA and lipid nanoparticles
- Regulatory requirements
- Viral variants
- Vaccine compliance.
Identification of the target antigen
Knowledge gained from these previous events enabled the rapid identification and release of the SARSCoV- 2 viral sequence in January 2020”
The initial challenge is to identify a relevant antigen target to encode in the vaccine that leads to a protective immune response. For effective vaccine design, we must identify emerging threats, understand the class of pathogen (such as its genome, structure and lifecycle) and determine its interaction with the host immune system. Recent technological advances, such as rapid genomic sequencing and other microbiological and molecular research tools, allow the rapid and extensive study of pathogen biology. These advances facilitate the identification of clinically important mutations that alter infectivity, severity, or immune susceptibility of pathogens. Fortunately, in the case of SARS-CoV-2, coronaviruses had been extensively studied as important human pathogens due to the outbreaks of the Severe Acute Respiratory Syndrome (SARS) in 2002/2003 and the Middle East Respiratory Syndrome (MERS) in 2012. Knowledge gained from these previous events enabled the rapid identification and release of the SARS-CoV-2 viral sequence in January 20207,8 just days after the first reported cases of pneumonia in Wuhan, China.
mRNA synthesis and purification
Once the sequence of the viral antigen is known, development of the vaccine can commence with the synthesis of the mRNA (Figure 1). To start, mRNA production is facilitated by enzymatic in vitro transcription (IVT), which uses a DNA template of the target, such as the CoV-2 spike protein. Initially, the template plasmid DNA is linearised using a restriction enzyme to allow synthesis of RNA transcripts. Following template linearisation, the mRNA is synthesised from nucleotide triphosphates (NTPs) by a DNA-dependent bacteriophage RNA polymerase. Once transcription is complete, the starting template DNA is digested with enzymes while the produced mRNA is purified to take forward for formulation and filling. It is possible to use traditional methods for the production of antigens such as the coronavirus spike protein, as is the case with the recombinant nanoparticle Novavax SARS-CoV-2 vaccine under development, which uses an engineered baculovirus containing a gene for a modified SARS-CoV-2 spike protein. However, the generation of the mRNA is easier than attempting to produce and purify the spike protein itself in a laboratory and subsequent manufacturing at scale. Also, the DNA template can be quickly modified, allowing rapid responses to variants as they develop.
However, mRNA synthesis and purification is not without its own challenges. As an emerging technology, a robust and compliant method of production has required rapid development and scale up. Larger scale production has also demonstrated a bottleneck from supply chain pressures with ever increasing demands placed on raw material requirements such as plasmid DNA, NTPs and formulation components in the current crisis.
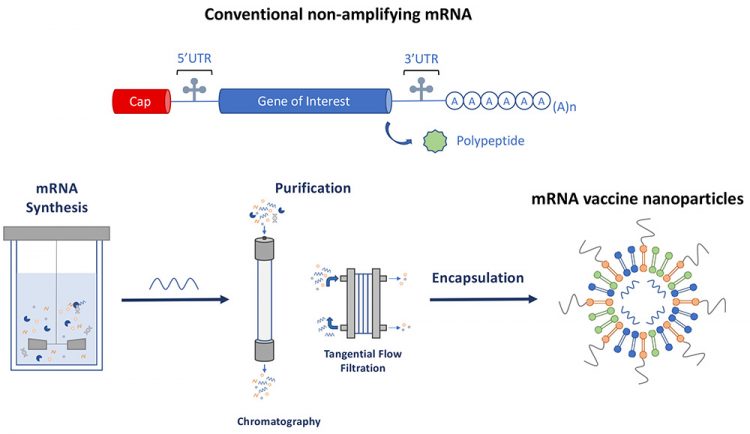
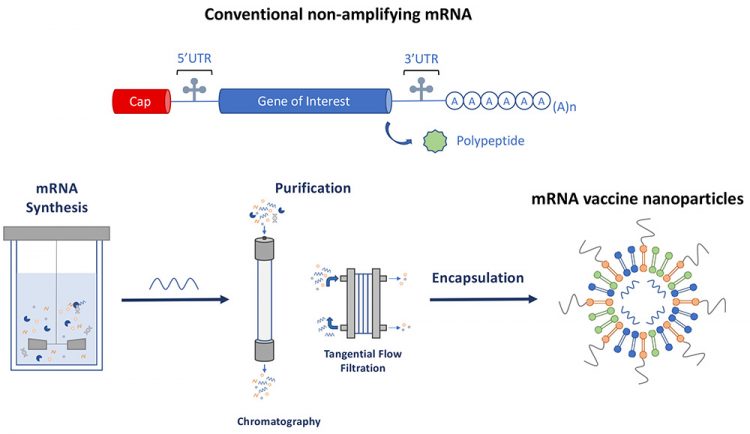
Figure 1: Schematic representation of conventional non amplifying mRNA and the production of mRNA vaccine nanoparticles.
mRNA Encapsulation
mRNA is a large hydrophilic molecule that does not naturally enter cells on its own, is unstable in the bloodstream and can undergo rapid degradation by hydrolysis of the mRNA polymer chain. Encapsulation is the process of creating lipid nanoparticles containing and protecting the mRNA. By encapsulating the mRNA in lipid nanoparticles, the mRNA is protected during storage, injection and transport through the blood stream. Additionally, since the lipid nanoparticles are lipophilic, they support delivery of the mRNA into cells.9
mRNA is a large hydrophilic molecule that does not naturally enter cells on its own”
To encapsulate mRNA, microfluidic mixing is used to combine the mixture of lipids dissolved in ethanol with the aqueous solution containing the mRNA. This mixing causes the lipids to form particles around the mRNA molecule in the condensation process.10 The typical structure of lipid nanoparticles therefore is an aqueous core containing the mRNA surrounded by a lipid bilayer shell. The majority of lipid nanoparticles use cationic lipids, cholesterol and lipid-anchored polyethylene glycol (PEG), which form complexes with the negatively charged mRNA molecule.3 However, the encapsulation process must be optimised for each different mRNA molecule, with different combinations and ratios of the lipids being required to achieve optimal lipid particle size for injection, encapsulation efficacy, and stability and mRNA stability.
Stability, formulation and analytical characterisation
During injection, the transient nature of the mRNA molecule is a benefit, preventing it from continually generating the antigen after the immune response has been triggered. The same property, however, is a downside for stability and storage. To facilitate global delivery, it is vital to ensure the mRNA is stabilised for prolonged periods prior to dosing of a patient. Since hydrolysis can cause the mRNA to break down or degrade when stored at elevated temperatures, the Pfizer-BioNTech and Moderna SARS-CoV-2 vaccines are stored at -70°C and -20°C respectively, to slow this process.11,12
Frustratingly, while low temperature storage in the frozen state can slow the degradation of the mRNA, it can have a detrimental effect on the physical stability of the lipid nanoparticles. During freezing, water forms ice crystals, which concentrates the nanoparticles and can lead to aggregation. To reduce the aggregation upon freezing and storage, sugars (eg, trehalose and sucrose) are added, which entrap the nanoparticles in an amorphous matrix which isolates them and reduces their mobility, which prevents damaging aggregation.
Another way to make mRNA vaccines more stable is to add stabilisers (sugars) and remove water from the product through a process called lyophilisation or freeze-drying. This has been shown to allow some mRNA vaccines to be stored in a refrigerator instead of a freezer. However, during the freezing step of the lyophilisation, the same aggregation process must be prevented.13
There are many unique challenges and issues associated with the use of lipid nanoparticle-encapsulated mRNA based vaccine”
The analytical testing required to ensure stability and efficacy is also a challenge for the development of lipid nanoparticle vaccines. Analysis of mRNA specific critical quality attributes (CQAs) is required, such as integrity, potency, capping efficiency, and process related residuals such as DNA. Additionally, assessment of CQAs associated with the lipid nanoparticle encapsulated mRNA are required, such as physicochemical properties, lipid content/composition and encapsulation efficiency. Fortunately, as lipid nanoparticles have been researched and developed for many years there are several testing methods available that can be applied. However, these methods require time to develop to ensure that they are suitable to support the development, characterisation and release testing of the specific product through its full lifecycle through to approval and commercial production.
Regulatory requirements
As with all drug products, regulatory guidance and rules must be followed. From a regulatory perspective, there is currently no specific ICH or FDA guidance for mRNA-based therapeutics. However, principles from the existing regulations can be applied such as FDA CFR Title 21 for guidance on Chemistry, Manufacturing and Controls requirements and ICH guidelines for development, stability, cGMP, method validations and setting specifications. Vaccines are unique in that they are usually administered to very large numbers of healthy people, thus safety and quality are paramount.6 Novel mRNA lipid nanoparticle vaccines are faced with additional regulatory challenges as there is limited historical knowledge of the technology and industry approval. Therefore, compared to more traditional vaccines, extra scrutiny is required to ensure they are safe and effective whilst the regulatory guidance specifically for mRNA-based therapeutics is generated.
Viral variants
Viruses continually mutate as they are transmitted, thus variants constantly emerge as demonstrated by the rising number of variants of SARS-CoV-2 that have emerged independently in many global strains, such as those from the UK, Brazil and India. If these variants have traits which help the virus become more transmissible, they will become the predominant strain.14 While the current vaccines still offer a high level of protection to the known new variants, it is possible that the virus may mutate in a region impacting the vaccines encoded antigen. If this occurs, the new variant may reduce the efficacy of existing vaccines,17 requiring the production of a new variant vaccine. This will require a rapid adaptation of the current technology so that future vaccine produced will contain mRNA targeting the new variant of concern. These potential variant vaccines come with the same challenges as the current vaccine but will need to be produced in a fraction of the time to ensure they are effective in stopping a second pandemic.
Vaccine compliance
As vaccines are distributed and administered throughout the UK and globally, there are individuals who may refuse to be vaccinated. They may not accept the vaccination due to perceived risks and dangers that are often spread through misinformation. The anti‑vaccine movement has been around for several decades, and was exacerbated after a researcher published a paper in 1998 stating that the measles‑mumps‑rubella (MMR) vaccines caused autism. This paper was officially labelled as fraudulent but it served to trigger debate over the safety of vaccines, which is ongoing today.15
The goal of the large-scale vaccination programme is to achieve herd immunity and it is therefore critical to achieve a high uptake of vaccines against the pathogen. In the case of SARS‑CoV-2 virus, it is estimated to require 60‑70 percent of the population being vaccinated to prevent spread and protect those who are vulnerable due to weakened immune systems or those who cannot be vaccinated for medical reasons.16 mRNA lipid nanoparticle vaccines have been through several clinical trials to prove their effectiveness and safety, and the continued rollout of the vaccines also adds to this body of evidence showing that the vaccines are safe. However, although the vaccines may be perfectly safe and have passed the same regulatory hurdles as other traditional vaccines, the rapid development of the novel mRNA lipid nanoparticle vaccines in a such a short time period means that may add to the distrust some individuals have regarding the use of vaccines.
Conclusion
Several COVID-19 vaccines have now been approved for use and many more are in varying stages of development and trials. There are many unique challenges and issues associated with the use of lipid nanoparticle-encapsulated mRNA based vaccine and although many have been overcome, there are still areas that could be improved and developed to enable vaccines of this type to be produced quickly, whilst remaining safe and effective against the SARS-CoV-2 virus and any emerging variants of concern.
About the authors
Andrew Bright joined CPI in November 2020 as Team Leader in Formulation and Fill, specialising in the formulation of liquid and freeze-dried biologics. Previously, he was a Senior Scientist at the Biopharma Group, where he developed freeze dried formulations and conducted freeze drying cycle optimisation and consultancy. He was also a Senior Scientist at Pfizer in liquid formulations, specialising in freeze dried formulation design, process development, and scale up. Andrew holds an MChem in Chemistry with a focus in Pharmaceutical and Forensic science and received his PhD from the University of Bradford where he investigated freeze dried vaccine formulations. His thesis was titled “Mechanistic Insights into the Stabilisation of Biopharmaceuticals Using Glycine Derivatives”.
Stuart Jamieson is Head of Research at CPI Biologics, and has almost 20 years’ experience in process development, scale up, characterisation and manufacture of biologics. His experience spans all phases of bioprocess development, and with a wide range of biopharmaceuticals including recombinant proteins, antibodies, viral vectors and vaccines. Stuart joined CPI in 2014 and now leads the technical team encompassing upstream, downstream, analytical and data sciences, working across mammalian, microbial, viral vector, nucleic acid and cell-free projects. His previous experience includes working in senior process development and technical leadership roles at Fujifilm Diosynth Biotechnologies, Merck & Co and Avecia Biologics. He has a degree in Biochemistry and a PhD in Structural Biology from The University of Sheffield.
References
- C. Romain Vuillemot – LIRIS, École Centrale de Lyon; Philippe Rivière – LIRIS, VisionsCarto; Pierre Ripoll – LIRIS, INSA Lyon; Julien Barnier – Centre Max Weber, “About Mapping, Living Guideus Living Mapping,” 2021. https://covid-nma.com/vaccines/mapping/ (accessed Mar. 08, 2021).
- P. Ball, “The lightning-fast quest for COVID vaccines — and what it means for other diseases,” 2021.
- P. P. G. Guimaraes et al., “Ionizable lipid nanoparticles encapsulating barcoded mRNA for accelerated in vivo delivery screening,” J. Control. Release, vol. 316, pp. 404–417, 2019, doi: 10.1016/j.jconrel.2019.10.028.
- A. Wolff et al , “Direct gene transfer into mouse muscle in vivo. Science,” Science (80-. )., no. 247, pp. 1465–1468, 1990.
- E. Jirikowski, G. F., Sanna, P. P., Maciejewski-Lenoir, D. & Bloom, “Reversal of diabetes insipidus in Brattleboro rats: intrahypothalamic injection of vasopressin mRNA,” Science, vol. 255, pp. 996–998, 1992. doi: 10.1126/science.1546298. PMID: 1546298
- Y. Granot and D. Peer, “Delivering the right message: Challenges and opportunities in lipid nanoparticles-mediated modified mRNA therapeutics—An innate immune system standpoint,” Semin. Immunol., vol. 34, pp. 68–77, 2017, doi: 10.1016/j.smim.2017.08.015.
- Y. Holmes, E. Zhang, “Novel 2019 coronavirus genome – SARS-CoV-2 coronavirus – Virological,” Virological.org, 2020. https://virological.org/t/novel-2019-coronavirus-genome/319.
- X. et al Zhou, P., Yang, XL., Wang, “A pneumonia outbreak associated with a new coronavirus of probable bat origin,” Nature, 579, pp. 270–273, 2020. https://doi.org/10.1038/s41586-020-2012-7
- K. Ita, “Coronavirus Disease (COVID-19): Current Status and Prospects for Drug and Vaccine Development,” Arch. Med. Res., vol. 52, pp. 15–24, 2021, doi: 10.1016/j.arcmed.2020.09.010.
- A. Rodríguez-Gascón, A. del Pozo-Rodríguez, and M. Á. Solinís, “Development of nucleic acid vaccines: Use of self-amplifying RNA in lipid nanoparticles,” Int. J. Nanomedicine, vol. 9, pp. 1833–1843, 2014, doi: 10.2147/IJN.S39810.
- “Information for Healthcare Professionals on Pfizer / BioNTech COVID-19 vaccine,” 2021. https://www.gov.uk/government/publications/regulatory-approval-of-pfizer-biontech-vaccine-for-covid-19/information-for-healthcare-professionals-on-pfizerbiontech-covid-19-vaccine (accessed June. 02, 2021).
- “Information for Healthcare Professionals on COVID-19 Vaccine Moderna,” 2021. https://www.gov.uk/government/publications/regulatory-approval-of-covid-19-vaccine-moderna (accessed Mar. 08, 2021).
- P. Zhao et al., “Long-term storage of lipid-like nanoparticles for mRNA delivery,” Bioact. Mater., vol. 5, pp. 358–363, 2020, doi: 10.1016/j.bioactmat.2020.03.001.
- J. W. Tang, P. A Tambyah, D. S. C. Hui, “Emergence of a new SARS-CoV-2 variant in the UK,” Journal of Infection, Volume 82, Issue 4, Pages e27-e28, 2021, https://doi.org/10.1016/j.jinf.2020.12.024
- M. C. Mills and J. Sivelä, “Should spreading anti-vaccine misinformation be criminalised?,” BMJ, vol. 372, pp. 1–3, 2021, doi: 10.1136/bmj.n272.
- A. Koirala, Y. Jin, A. Khatami, C. Chiu, and P. N. Britton, “Vaccines for COVID-19: The current state of play,” Paediatric Respiratory Reviews, Volume 35, Pages 43-49, 2020. https://doi.org/10.1016/j.prrv.2020.06.010
- Episode #20 – COVID-19 – Variants & Vaccines. https://www.who.int/emergencies/diseases/novel-coronavirus-2019/media-resources/science-in-5/episode-20—covid-19—variants-vaccines
Issue
Related topics
Biologics, Bioproduction, Drug Delivery Systems, Drug Development, Drug Safety, Drug Targets, Immunisation, mRNA, QA/QC, Regulation & Legislation, Research & Development (R&D), Vaccine Technology, Vaccines, Viruses
Related drugs
Related diseases & conditions
Coronavirus, Covid-19, Middle East Respiratory Syndrome (MERS), Severe Acute Respiratory Syndrome (SARS)