Particle characterisation in drug delivery
Posted: 5 September 2014 |
The use of materials in nano-scale dimensions is proving to be a promising approach to overcome drug delivery challenges. ‘Nanomedicine’ technologies are gradually achieving commercial success and reaching the clinic. Sub-micron nanocarriers have the potential to ferry the therapeutic to its site of action and in this process overcome the biological barriers and achieve targeted drug delivery, controlled or stimuli-responsive delivery and protect the therapeutic from biological milieus.
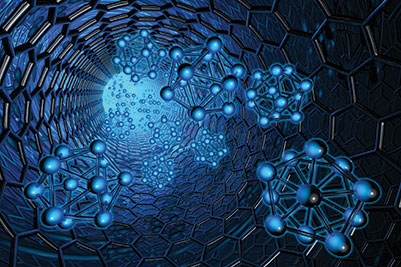
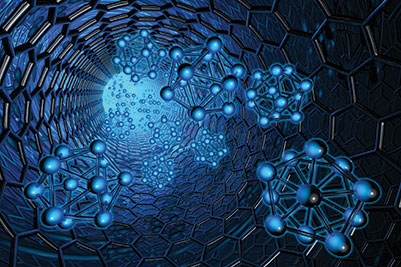
Many different types of nanocarriers have been described, including polymeric nanoparticles (NPs), liposomes, solid lipid NPs, micelles, dendrimers and metal NPs among other systems (the terms ‘nanomedicine’, ‘NP’ and ‘nanocarriers’ are used herein to describe all nanosystems). Of particular interest are nanocarriers with the ability to act selectively and target cell internalisation processes, guiding the therapeutic into subcellular regions. NP features important in dictating their drug delivery performance, including targeted delivery and cellular trafficking, are their size, shape and surface characteristics such as surface charge, chemistry and the distribution of ligands.
Why NP characterisation matters in drug delivery
NPs can be fabricated from different materials with different physical and chemical properties. In addition, they can be surface decorated with a range of ligands in order to enhance their therapeutic response (e.g. improving targeting or reducing immune response) or chemical groups that improve NPs stability. As a result, a huge number of combina tions of NP parameters are possible, with the idea of tailoring these systems for specific applications. As mentioned above, the interdependent effect of NP size, shape, composition and surface chemistry is crucial in dictating their interaction with cells and therefore drug delivery capability. Considering NP size, it has been shown that this parameter affects the cell uptake efficiency and kinetics. Size-dependent uptake in different cell lines has been observed for metal, mesoporous silica and polystyrene NPs9, with the maximum efficiency at a NP core size in the range of 30 50nm10. The effect of size on NP cell uptake and also transepithelial transport was also shown for systems displaying ligands targeting transcytosis systems. NP size also affects tissue distribution and elimination, as well as cytotoxicity. Surface charge is also an important factor determining the fate of NP interaction with the biological systems. In terms of cell uptake, positively charged NP penetrate the cell membranes and internalisewith the greatest efficiency compared to neutral and negatively charged particles, probably due to binding to negatively charged groups on the cell surface. However, positively charged particles are also considerably more toxic compared to negatively charged systems. Clearly, the biological response of nanomedicines relies heavily on their specific physicochemical characteristics. This is important as for this class of therapeutics the biological effect generated depends not only on the action of the active therapeutic(s) incorporated within the system but also on the characteristics of other components such as nanocarrier size. In fact, even a small variation in physicochemical characteristics of the system may have a significant impact on the performance of the nanomedicine. Accurate and detailed characterisation of these systems is therefore critical in the development and clinical use of nanomedicines to ensure safe use and reproducibility.
Characterisation of NP size
There are several methods available to determine NP size and some of these will be discussed further on (for a more comprehensive review on the topic, the reader is referred to15). Note that each technique has its strengths and weaknesses, which have to be considered when characterising NPs. The application of a particular method depends on many factors, including the anticipated NP size range, mono- and polydispersity and NP material. Overall, it is recommended that different NP sizing methods are employed to ensure robustness, especially when considering the importance on biological response mentioned above.
Dynamic light scattering (DLS)
DLS is one of the most commonly used techniques for particle size measurement. This measures the hydrodynamic diameter of a particle via a laser beam that is scattered by the NPs in a suspension or solution. The Brownian motion of particles causes random fluctuations in the intensity of the scattered light around a mean value, used to determine the particle diffusion coefficient, which is related to its hydrodynamic radius via the Stokes–Einstein relationship15.
DLS is typically used to measure NPs in the 1nm-500nm range, although some systems claim a range of 0.3nm-10µm. While a user-friendly technique, the analysis of multimodal particle size distribution is problematic with DLS16. This is because the results are strongly biased in the presence of a small fraction of large particles as the signal intensity of a spherical particle with a radius r is proportional to its diameter to the power of 6. To exemplify, when a mixture of 5 and 50nm NPs is measured, the signal of 5nm particles is masked by that of 50nm NPs, with the latter scattering 106 as much light. Consequently, average particle size values determined by DLS are biased. DLS therefore is best suited to measure the size of monodisperse NP samples.
Electron microscopy (EM)
EM uses electron beams to visualise NPs. The use of EM is advantageous because it provides information on size and shape (morphology) in one analysis by direct visualisation. In scanning electron microscopy (SEM) electrons come from the sample surface, whereas in transmission electron microscopy (TEM) electrons pass through the sample. TEM has a superior resolution to SEM and is usually employed to study NP morphology. Due to image contrast, TEM is most suitable for particles containing heavier atoms such as gold and silver NPs. TEM, however, is a laborious technique and therefore is not suited to routine size measurements of large numbers of NP samples, but is usually reserved for morphological characterisation.
Differential Centrifugal Sedimentation (DCS)
DCS is based on the principle that with particles of the same density, larger particles sediment faster than smaller particles. Although most NPs do not sediment through simple gravitation (due to small size), their sedimentation can be induced by centrifugation. The disc centrifuge contains a hollow, optically clear disc, which has a central opening. The disc rotates at a known speed ranging from 600-24000RPM. The empty spinning disc chamber is partly filled with liquid, which forms a liquid ring with a density gradient. The central opening is used to inject the sample for analysis. DCS gives a very high resolution and different nanoparticulate species of <5% difference in diameter can be resolved completely. However, analysis times for smaller particles (<50nm) are long.
DCS has recently been used to measure the change in size of gold NPs by changing ligand shells17. For gold–polyamide functionalised NPs, a shift of 0.5nm in the particle diameter was reported following functionalisation and in case of NP functionalisation with a larger molecular weight entity, namely single stranded DNA, this shift amounted to 2.1nm. This is therefore an example of the high-resolution NP sizing offered by this technique, which can be used to characterise NP, even following functionalisation or surface decoration with species with slight variations in molecular weight. The use of DCS to measure the size of ligand modified gold NPs was also recently reported Krpetic et al.18. In this study, ligand shells were composed of polyethylene glycol-substituted alkane thiol of different chain length, or oligopeptides. DCS was highly sensitive to small changes in the thickness of particles due to the change in ligand shell, successfully measuring variations in the thickness of ligand shells on particles amounting to as little as 0.1nm.
Nanoparticle Tracking Analysis (NTA)
NTA is a technology that enables simultaneous visualisation and multi-parameter analysis of NPs, relating the rate of Brownian motion to particle size. The particles are visualised based on the light they scatter when illuminated by laser light. Unlike DLS, this technique has the ability to visualise individual particles in solution (each scattering source is tracked separately), then analyse for size (determined using the Stokes–Einstein equation) and count/concentration, both under scatter and fluorescence mode. Counting individual particles is useful when dealing with polydisperse samples, although NTA is still unable to separate fractions of particles with relative size difference of less than 50%. Filipe et al. compared NTA with DLS using polystyrene beads19 and reported that the main advantage of NTA is its unbiased peak resolution of polydisperse samples, which was not possible to achieve with DLS.
Characterisation of NP surface
Surface charge: Zeta (ζ) potential
The surface charge of NPs is very important in drug delivery because it influences the interaction with the biological system, as well as the interaction with other species (including neighbouring NPs) in the suspension. Measurement of the ζ potential provides information on the net charge a NP has and therefore gives an indication of the electrostatic charge repulsion or attraction between particles in a liquid suspension. The ζ potential is usually measured by application of an electric field to the sample and measuring the velocity at which charged species move toward the electrode15. The ζ potential is generally a good measure of the stability of particles in the suspension as a large repulsion (high ζ potential) between particles means that they will stay away from each other (less chance of aggregation), which is generally aimed for nanosystems in drug delivery, and with weaker repulsion the likelihood of particles coming together (producing aggregates) is higher.
The charge on the surface of particles and properties of the suspension diluent determine the zeta potential. The change in pH and the ionic strength of diluent will affect particle ζ potential. The ζ potential measurements are therefore highly sensitive to sample suspension properties and can be used to determine optimum dispersion protocols and to study the stability of samples under changing conditions. It is recommended that ζ potential measurements of NPs are conducted in relevant biological media/solutions simulating the environment within which they interact with the biological systems.
Surface chemistry
The importance of NP surface chemistry in determining their biological response is increasingly being recognised, although the area remains somewhat poorly understood. A number of different surface-analysis techniques can be useful for NP surface characterisation, including electron spectroscopies (Auger electron spectroscopy [AES] and X-ray photoelectron spectroscopy [XPS], the latter possibly being the most widely used surface spectroscopy), ion-based methods (secondary ion mass spectrometry [TOF-SIMS] and low energy ion scattering [LEIS]) and scanning probe microscopy (atomic force microscopy [AFM] and scanning tunnelling microscopy [STM]). For a comprehensive review on the usefulness of these techniques in NP surface chemical analysis the reader is referred to20.
NP changes within the biological environment
In a complex biological environment nanocarriers may undergo a complete transformation in terms of size and surface characteristics. The colloidal stability of NP is influenced by many factors such as the ionic strength, pH and composition of the solution. The formation of a protein corona, which consists of plasma proteins such as albumin as a major component21-24, may significantly alter NP characteristics, including size and surface charge (and therefore the likelihood of aggregation). This in turn may completely change the nanocarrier’s biocompatibility and biodistribution25-26, as well as targeting capacity27. The behaviour of nanomedicines in the body is therefore an important point to consider when designing such systems as there is little point in formulating complex nanocarriers, for example with defined size and controlled surface distribution of targeting moieties/ligands, when such ‘designer’ nanocarriers morph into something entirely different in a biological environment. This point has recently been demonstrated with transferrin-functionalised NPs, the targeting ability of which disappears in a biological environment due to a biological media-originating surface-adsorbed protein corona27.
With the aforementioned point in mind, characterisation of NPs should be conducted using appropriate techniques and for multiple parameters. This characterisation should be performed under physiologically relevant conditions reflecting the biological environment of the body in which the nanosystem is both distributed and exerts its therapeutic action.
Conclusion
The promise of nanomedicine has placed the development of cutting-edge nanosystems at the forefront of drug delivery research. The technology available to analyse NPs has improved considerably, which will no doubt advance our understanding of the influence of NP properties on their biological activity, as well as improve our ability to translate these systems into the clinic. Unfortunately, the science behind nanotechnology is much more complex than for small molecules and not all researchers developing various nanosystems will have all the tools enabling full characterisation in terms of size, shape, charge and surface chemistry, which is crucial as these properties govern the biological response of nanomaterials.
Furthermore, this characterisation should take place within a biologically relevant environment. There is currently no single technique that can provide simultaneous information on all of these parameters (apart from instrumentation measuring both size and ζ potential), and even for determination of a single parameter such as size, different techniques exist, the use of which has to be carefully considered depending on the NP sample. The availability of instrumentation that can provide reliable multiparameter information, combined with ability to accommodate a range of different nanosystems (e.g. wide NP size range) and easy operation for users from different disciplines is highly desirable.
References
- D. Vllasaliu, R. Fowler and S. Stolnik, Expert Opin Drug Deliv, 2014, 11, 139-154.
- D. Vllasaliu, C. Alexander, M. Garnett, M. Eaton and S. Stolnik, J Control Release, 2012, 158, 479-486.
- R. Fowler, D. Vllasaliu, F. H. Falcone, M. Garnett, B. Smith, H. Horsley, C. Alexander and S. Stolnik, J Control Release, 2013, 172, 374-381.
- L. Y. Chou, K. Ming and W. C. Chan, Chem Soc Rev, 2011, 40, 233-245.
- A. Malugin and H. Ghandehari, J Appl Toxicol, 2010, 30, 212-217.
- B. D. Chithrani, A. A. Ghazani and W. C. Chan, Nano Lett, 2006, 6, 662-668.
- J. Huang, L. Bu, J. Xie, K. Chen, Z. Cheng, X. Li and X. Chen, ACS Nano, 2010, 4, 7151-7160.
- F. Lu, S. H. Wu, Y. Hung and C. Y. Mou, Small, 2009, 5, 1408-1413.
- J. A. Varela, M. G. Bexiga, C. Aberg, J. C. Simpson and K. A. Dawson, J Nanobiotechnology, 2012, 10, 39.
- L. Shang, K. Nienhaus and G. U. Nienhaus, J Nanobiotechnology, 2014, 12, 5.
- R. Fowler, D. Vllasaliu, F. F. Trillo, M. Garnett, C. Alexander, H. Horsley, B. Smith, I. Whitcombe, M. Eaton and S. Stolnik, Small, 2013, 9, 3282-3294.
- M. Cho, W. S. Cho, M. Choi, S. J. Kim, B. S. Han, S. H. Kim, H. O. Kim, Y. Y. Sheen and J. Jeong, Toxicol Lett, 2009, 189, 177-183.
- T. H. Kim, M. Kim, H. S. Park, U. S. Shin, M. S. Gong and H. W. Kim, J Biomed Mater Res A, 2012, 100, 1033-1043.
- A. Verma and F. Stellacci, Small, 2010, 6, 12-21.
- K. E. Sapsford, K. M. Tyner, B. J. Dair, J. R. Deschamps and I. L. Medintz, Anal Chem, 2011, 83, 4453-4488.
- X. Y. Lu, D. C. Wu, Z. J. Li and G. Q. Chen, Prog Mol Biol Transl Sci, 2011, 104, 299-323.
- Ž. Krpetić, I. Singh, W. Su, L. Guerrini, K. Faulds, G. A. Burley and D. Graham, Journal of the American Chemical Society, 2012, 134, 8356-8359.
- Z. Krpetic, A. M. Davidson, M. Volk, R. Levy, M. Brust and D. L. Cooper, ACS Nano, 2013, 7, 8881-8890.
- V. Filipe, A. Hawe and W. Jiskoot, Pharm Res, 2010, 27, 796-810.
- D. R. Baer, D. J. Gaspar, P. Nachimuthu, S. D. Techane and D. G. Castner, Anal Bioanal Chem, 2010, 396, 983-1002.
- M. S. Ehrenberg, A. E. Friedman, J. N. Finkelstein, G. Oberdorster and J. L. McGrath, Biomaterials, 2009, 30, 603-610.
- R. Gref, M. Luck, P. Quellec, M. Marchand, E. Dellacherie, S. Harnisch, T. Blunk and R. H. Muller, Colloids and Surfaces B-Biointerfaces, 2000, 18, 301-313.
- M. Lundqvist, J. Stigler, G. Elia, I. Lynch, T. Cedervall and K. A. Dawson, Proc Natl Acad Sci U S A, 2008, 105, 14265-14270.
- E. Casals, T. Pfaller, A. Duschl, G. J. Oostingh and V. Puntes, Acs Nano, 2010, 4, 3623-3632.
- J. C. Leroux, F. Dejaeghere, B. Anner, E. Doelker and R. Gurny, Life Sciences, 1995, 57, 695-703.
- S. Stolnik, L. Illum and S. S. Davis, Advanced Drug Delivery Reviews, 1995, 16, 195-214.
- A. Salvati, A. S. Pitek, M. P. Monopoli, K. Prapainop, F. B. Bombelli, D. R. Hristov, P. M. Kelly, C. Aberg, E. Mahon and K. A. Dawson, Nature Nanotechnology, 2013, 8, 137-143.
Biography
Dr Driton Vllasaliu is a Senior Lecturer at the School of Pharmacy, University of Lincoln. He is a Pharmacist and obtained his PhD in Drug Delivery from the University of Nottingham in 2010. He also works on improved cell models of human mucosal tissues as a means to study drug-delivery systems.
Ishwar Singh is a Senior Lecturer in Biological Chemistry at the School of Pharmacy, University of Lincoln. Prior to Lincoln, he had held many prestigious fellowships such as the Alexander von Humboldt fellowship, Germany; and Senior Research Fellowship, CSIR, India. He is an organic chemist. He has developed bioconjugations for DNA, RNA and polymer modifications in water. He is currently leading research in Biologics delivery, Peptides, Sequence selective DNA cross linking, Nanoparticles modifications for drug delivery applications and broad spectrum antibiotics.