Indirect modulation of cardiac ion channels and implications for preclinical safety assessment
Posted: 19 August 2010 |
The preclinical assessment of a small molecule’s liability for QT interval prolongation is an essential part of the drug discovery process. Patch clamp assays for heterologously expressed recombinant cardiac ion channels are widely used in the pharmaceutical industry to evaluate potential drug-channel interactions. These assays are generally acute assessments and are not designed to detect indirect channel modulations that may result in QT prolongation. Despite the abundant literature demonstrating potential transcriptional, translational and post-translational mechanisms for indirect ion channel modulation, contribution of these mechanisms to drug-induced QT prolongation and/or arrhythmia propensity is not well understood. In this brief review, we discuss some potential mechanisms through which indirect ion channel modulation can produce QT prolongation and strategies for their early detection and mitigation.
Ion channels play essential roles in excitation, contraction and repolarisation of the heart1. Given their fundamental contributions to cardiac electrical activity, it is not surprising that mutations in the genes encoding various cardiac ion channels result in multiple cardiac arrhythmia syndromes, some of which are associated with prolongation of the QT interval and sudden cardiac death2. In particular, drug-induced inhibition of IKr, the rapid component of the delayed rectifier potassium current (a.k.a. hERG current), has been linked to acquired long QT syndrome Type 2 (LQT2) and potentially fatal arrhythmias in humans2-5.
In the last two decades, several drugs were withdrawn from the market or received black box FDA labelling due to reported clinical cases of QT interval prolongation, ventricular arrhythmias and sudden death. Other drugs were denied regulatory approval due to their potential for QT prolongation5,6. Furthermore, off-target activity of drugs on cardiac ion channels has been shown to be associated with increased mortality in patients with underlying cardiovascular diseases7. In light of the increasing importance of ion channel interactions for cardiac safety, regulatory authorities published guidelines for preclinical (International Conference on Harmonisation, ICH S7B) and clinical assessment (ICH E14) of QT interval prolongation. Since clinical arrhythmia risk is a major criterion for compound termination, preclinical profiling for off-target cardiac ion channel interactions early in the drug discovery process has become common practice in the pharmaceutical industry8,9.
The first step in the preclinical evaluation of small molecule QT prolongation liability is an in vitro assessment of ion channel activity based on patch clamp electrophysiology studies in recombinant expression systems. This is typically followed by an in vivo telemetry study in non-rodent species8,9. Patch clamp assays have been extensively validated, and recent technological advances in automated electrophysiology systems have allowed their implementation in the early lead optimisation stage of drug discovery to help identify potential cardiac liabilities. However, patch clamp assays using heterologously expressed recombinant channels are acute functional assessments and are best used to detect direct, rather than indirect, modulation of the channel protein.
Indirect modulation of cardiac ion channels may significantly contribute to QT interval prolongation and help explain discrepancies between in vitro and in vivo data10,11,12. One of the most studied examples of indirect channel modulation is drug-induced inhibition of hERG channel trafficking to the plasma membrane13,14. hERG channel trafficking inhibition has been proposed as a contributing mechanism for the delayed QT interval prolongation observed with arsenic trioxide and pentamidine treatments in animals and humans15,16. There are numerous other possible mechanisms (e.g., transcriptional, translational and post-translational) that could influence expression and functional properties of cardiac ion channels.
Despite copious literature indicating potential mechanisms for indirect ion channel modulation, contribution of these mechanisms to QT interval prolongation and/or arrhythmogenesis is not well understood. In this brief review, we discuss selected indirect channel modulation mechanisms, their link to QT prolongation, and potential pre-clinical strategies for early detection. We focus on ventricular repolarisation, a critical process in cardiac electrical activity during which irregularities can result in dangerous clinical arrhythmias. Special attention is given to in silico hypotheses for non-hERG off-target interactions based on predictive chemogenomic methods and how they can help the design of follow-up studies. Cardiac ion channel trafficking is not covered and readers are referred to recent review articles13,14.
Effects of glucose concentration on ventricular repolarisation
Changes in blood glucose levels have been shown to prolong the heart rate corrected QT interval (QTc) and, under certain circumstances, cause ventricular arrhythmias in healthy subjects and dogs17-19. Type I and II diabetes are also associated with increases of both QT interval duration and dispersion in patients of both genders and a wide range of age groups20-22. QTc prolongation and ECG abnormalities occur during nocturnal hypoglycaemia in patients with type 1 diabetes and investigators suggest these findings may help explain the ‘dead in bed’ syndrome18. Diabetes is strongly associated with sympatho – adrenal neuropathy, which may be responsible for increased QTc and QT dispersion due to elevated plasma concentrations of catecholamines seen during hypo- and hyperglycemia episodes23. However, conflicting data exists, as QTc prolongation was also seen in diabetic patients without autonomic neuropathy24. In studies of identical twins, QTc prolongation did not correlate with duration of diabetes or autonomic dysfunction24. Additionally, insulin levels had very little influence on QT dispersion25.
The hERG channel is modulated by extracellular glucose levels and it is plausible that impairment of hERG function may contribute to the ECG changes observed in hypo- and hyperglycemia26. Zhang et al studied the hERG channel in HEK 293 cells and showed that IKr amplitude decreased in response to extracellular glucose concentration changes in both directions26. The authors proposed that intracellular ATP generated by glucose metabolism acted as a substrate for protein kinase mediated channel phosphorylation and was therefore essential for the normal function of the channel. When hypoglycemia was reproduced by complete inhibition of glycolysis and oxidative phosphorylation by 2-deoxy-D-glucose substitution for glucose, IKr was diminished due to the depletion of intracellular ATP. Hyperglycemia again decreased IKr by overproduction of reactive oxygen species (ROS), by-products of oxidative glucose metabolism. Superoxide anion (O2 -) is the major ROS produced by hyperglycemia27,28 and acute elevations in glucose levels also depresses cells natural antioxidant defenses29. Excessive ROS decrease ATP production by inhibiting glycolysis and may contribute to the hERG channel inhibition in hyperglycemia30,31. In addition to their effects on IKr, hypo- and hyperglycemia can also potentiate the effects of IKr blockers such as dofetilide, further increasing their QT prolonging effects and the risk of proarrhythmia32.
In healthy subjects, glucose ingestion and subsequent increased plasma insulin levels has been shown to cause ECG changes including increased QT dispersion33. In patients with congenital long QT syndrome, insulin-induced QT interval prolongation was associated with T wave changes, suggesting that glucose-induced insulin secretion can influence repolarisation34,35. Although serum K+ levels were unchanged in this study, it is possible that insulin decreases K+ concentration in the extracellular space, thereby activating the Na+/K+ pump and hyperpolarising the transmembrane potential36. Insulin has also been shown to stimulate the L-type Ca2+ current in isolated rat ventricular myocytes in a concentration dependent manner37.
Overall, these investigations clearly implicate a role for plasma glucose and insulin levels in modulating cardiac ion channels and ventricular repolarisation. During drug development, these effects may not be detected until in vivo studies or, in some cases, until clinical studies because routine patch clamp studies are not designed to screen for indirect effects.
Plasma potassium levels and QTc
Serum K+ levels play a key role in the ventricular repolarisation: hypokalemia increases the cardiac action potential duration and prolongs QTc by decreasing IKr11,38. Initially the effects of low extracellular K+ were attributed to the accelerated inactivation of the channel39-41. However, more recent studies also suggested that extracellular K+ controls cell surface density of the hERG channel by modifying its gating state42,43. Massaeli et al showed that in low extracellular K+ media, hERG channels entered a novel nonconducting state, which was different from the inactivated or drug-blocked state. The nonconducting channels were subsequently internalised and degraded, decreasing the number functional channels42.
In humans, hypokalemia can occur secondary to numerous drug treatments or disease processes, and is a major risk factor for arrhythmia11,44. Furosemide is a loop diuretic and by inhibiting the Na-K-2Cl symporter in the renal loop of Henle, it can cause electrolyte imbalances (e.g. hypokalemia and hypo – magnesaemia) even at moderate doses, and thus produce QTc prolongation both in man and dogs. This highlights the critical role serum K+ levels play in modulation of cardiac ion channels and ventricular repolarisation11,41,44. Analogous to the furosemide scenario, small molecules could affect ventricular repolarisation indirectly by producing hypokalemia; they would appear clean in patch clamp studies but cause QT interval prolongation in vivo.
Effects of autonomic tone on ventricular repolarisation
Drugs could also indirectly induce QTc prolongation by influencing autonomic tone in isolation or in combination with other direct or indirect ion channel effects45-47. Ventricular arrhythmias are occasionally precipitated by physical or emotional stress suggesting a link between increased adrenergic stimulation and cardiac ion channel activity48. IKs has been shown to be regulated by α and β adrenergic stimulation via protein kinase A and C, which may explain the increased incidence of ventricular arrhythmias in LQTS-1 patients49. It has been shown that hERG/IKr is inhibited by α and β adrenergic stimulation which may be of importance in LQTS-2 where hERG currents are already impaired due to underlying gene mutations48,50-52. Chen et al have recently reported post-translational regulation of the hERG channel that may pertain to the electrical remodelling observed in chronic cardiac diseases53. In their experiments, prolonged α adrenergic stimulation influenced the balance of hERG channel synthesis and degradation via multiple signalling pathways involving PKC and PKA. There are also intriguing examples from clinical medicine linking autonomic mechanisms with cardiac repolarisation. A recent clinical report linked elevated catecholamines to profound ECG repolarisation changes in acute states of myocardial stunning, mimicking myocardial infarction54. Smith et al investigated how autonomic blockade might affect QT prolongation and found that autonomic block in the presence of the IKr blocker ibutilide resulted in exaggeration of drug-induced QT prolongation55, further strengthening the connection between autonomic modulation and repolarisation. In sum, there is a growing body of evidence establishing the importance of autonomic effects in cardiac safety.
In silico hypotheses for non hERG off-targets, based on predictive chemogenomics methods and how it can help design of follow-up studies
Predicting compound activity against a single target has always been a major objective in the field of cheminformatics. A substantial quantity of in vitro activity data has accumulated in public (Pubchem, chEMBL) and commercial (GVK, Wombat) chemogenomics databases over recent years. The systematic annotation of assays, targets, compounds and activities in these databases has allowed for large scale modelling and poly-pharmacology predictions that use chemical structure alone to rank the order of the most likely targets that might be modulated by a compound56. These predictions could be viewed as an inexpensive and rapid method for generating preliminary off-target interaction hypotheses when a candidate molecule possesses a QTc prolongation liability. Analysing model predictions for overlap with biologically relevant targets (as described above) that might explain the QTc liability or predict other potential safety risks would be the natural extension of this dataset and its most relevant application. Any identified overlaps or safety risks could then drive the design of future studies to elucidate mechanisms or mitigate risks. Since model predictions are based on published in vitro data, most follow up assays to confirm in silico off-target hypotheses should be available for investigative studies57.
Beyond direct cardiac ion channel interactions
In summary, several complex mechanisms including modulation of cardiac ion channels and autonomic tone may influence ventricular repolarisation and these factors should be taken into consideration when interpreting in vivo results45. In vitro patch clamp assays using heterologously expressed recombinant channels are widely used in the pharmaceutical industry during drug development. Modern automated electrophysiology technologies have enabled early phase, large scale implementation of these assays, allowing the screening of thousands of compounds. These assays, however, are likely to detect only direct modulation of channel proteins. Although pivotal to early screening, cardiac safety issues may stem from several other factors beyond direct cardiac ion channel modulation, as discussed above.
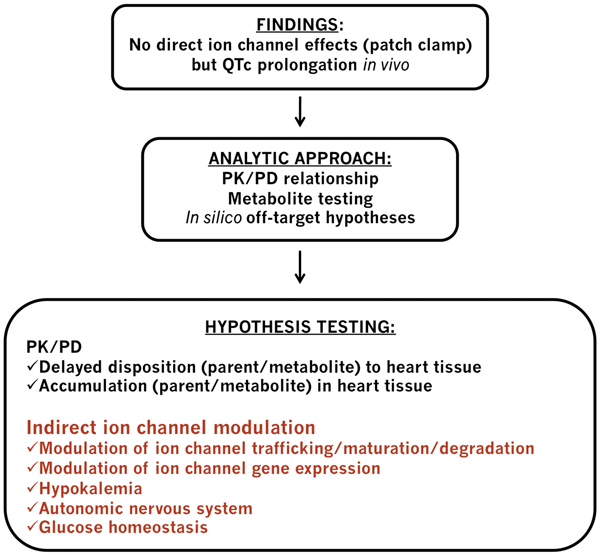
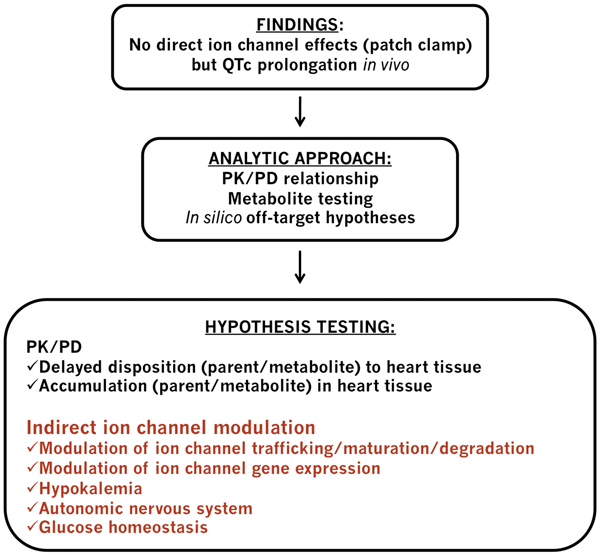
Figure 1 Cardiac safety schematic integrating indirect ion channel modulation analysis
A schematic of how indirect ion channel modulation might fit into an overall drug development safety analysis strategy is illustrated in Figure 1 above. In a chronic in vivo model, if QTc prolongation is seen for a late stage compound that does not directly block cardiac ion channels, human metabolites could be synthesised and tested in patch clamp assays. Determining the concentrations of the parent compound and its metabolite(s) in cardiac tissue together with pharmacokinetic/ pharmacodynamic (PK/PD) modelling of QTc prolongation could be instrumental in understanding the mechanisms involved58. Predictive in silico methods can be helpful to propose off-target hypotheses that could be tested in relevant in vitro assays. For example, cardiac ion channel trafficking assays could be used to test whether the compound blocks the channel trafficking to the surface membrane59. An important point to consider is that trafficking assays testing the channel protein expression on the cell surface may fail to identify compounds affecting channel maturation or degradation. In such cases, follow up patch clamp assays using longer incubation with the compound of interest may be implemented.
Additional pre-clinical assays could be run on a case-by-case basis at various stages of the process, including after the compound is tested in man. If the mechanism of effect is identified, relevant assays could be incorporated into the discovery phase for back up activities to try to remove the liabilities. This approach is likely to reduce the chances of having to halt progression of a compound late in the discovery phase after considerable resource investments. Early detection of these effects using in vitro methods, however, is challenging and the translatability of these effects from in vitro to in vivo and preclinical species to human is not yet well defined either.
It is vital to recognise that unexpected QTc prolongation could occur in preclinical telemetry studies or in human trials even with compounds showing no known direct effects on cardiac ion channels. A prudent strategy to avoid such unpleasant findings may be to invest more time and resources early in candidate profiling to identify the potential mechanisms responsible for QTc prolongation and design targeted experiments to help clarify impact or even abort development. A notable caveat to this strategy, however, is that the degree of safety risk incurred due to indirect cardiac ion channel modulation is usually difficult to quantify. Moreover, in vitro assays to characterise each potential mechanism with sufficient specificity and throughput may not always be available. Developing tools to accurately predict cardiac arrhythmia risks during drug development continues to challenge our field, whether addressing direct or indirect ion channel modulation. Awareness that indirect mechanisms can have a meaningful impact on safety is an important step forward.
References
- Nerbonne JM, Kass RS (2005) Molecular physiology of cardiac repolarization. Physiol Rev 85:1205-1253
- Chiang CE, Roden DM (2000) The long QT syndromes: Genetic basis and clinical implications. J Am College Cardiol 36: 1-12.
- Mitcheson JS, Chen J, Lin M, Culberson C, Sanguinetti MC (2000) A structural basis for drug-induced long QT syndrome. Proc Nat Acad Sci 97: 12329-12333.
- Sanguinetti MC, Jiang C, Curran ME, Keating MT (1995) A mechanistic link between an inherited and an acquired arrhythmia: hERG encodes the IKr potassium channel. Cell 8:299-307.
- van Noord C, Sturkenboom MCJM, Straus SMJM, Witteman JCM, Stricker BHCh (2010) Non-cardiovascular drugs that inhibit hERG-encoded potassium channels and risk of sudden death. Heart
- Shah RR (2005) Drug-induced QT interval prolongation–regulatory guidance and perspectives on hERG channel studies. Novartis Found Symp 266:251-280.
- Echt DS, Liebson PR, Mitchell LB, Peters RW, Obias-Manno D, Barker AH, Arensberg D, Baker A, Friedman L, Greene HL, et al. (1991) Mortality and morbidity in patients receiving encainide, flecainide, or placebo. The Cardiac Arrhythmia Suppression Trial. N Engl J Med 324:781-8.
- Pollard CE, Abi Gerges N, Bridgland-Taylor MH, Easter A, Hammond TG, Valentin JP (2010) An introduction to QT interval prolongation and non-clinical approaches to assessing and reducing risk. Br J Pharmacol 159:12-21.
- Sager PT (2008) Key clinical considerations for demonstrating the utility of preclinical models to predict clinical drug-induced torsades de pointes. Br J Pharmacol 154:1544-9.
- Au WY, Kwong YL (2008) Arsenic trioxide: safety issues and their management. Acta Pharmacol Sin 29:296-304.
- Chvilicek JP, Hurlbert BJ, Hill GE (1995) Diuretic-induced hypokalaemia inducing torsades de pointes. Can J Anaesth 42:1137-9.
- Kuryshev YA, Ficker E, Wang L, Hawryluk P, Dennis AT, Wible BA, Brown AM, Kang J, Chen XL, Sawamura K, Reynolds W, Rampe D (2005) Pentamidine-induced long QT syndrome and block of hERG trafficking. J Pharmacol Exp Ther 312:316-23.
- Dennis A, Wang L, Wan X, Ficker E (2007) hERG channel trafficking: novel targets in druginduced long QT syndrome. Biochem Soc Trans. 35:1060-3.
- Van der Heyden MA, Smits ME, Vos MA (2008) Drugs and trafficking of ion channels: a new pro-arrhythmic threat on the horizon? Br J Pharmacol 153:406-9.
- Ficker E, Kuryshev YA, Dennis AT, Obejero-Paz C, Wang L, Hawryluk P, Wible BA, Brown AM (2004) Mechanisms of arsenic-induced prolongation of cardiac repolarization. Mol Pharmacol 66:33-44.
- Eisenhauer MD, Eliasson AH, Taylor AJ, Coyne PE Jr, Wortham DC (1994) Incidence of cardiac arrhythmias during intravenous pentamidine therapy in HIV-infected patients. Chest 105:389-95.
- Eckert B, Agardh CD (1998) Hypoglycaemia leads to an increased QT interval in normal men. Clin Physiol 18:570-5.
- Gill GV, Woodward A, Casson IF, Weston PJ (2009) Cardiac arrhythmia and nocturnal hypoglycaemia in type 1 diabetes–the ‘dead in bed’ syndrome revisited. Diabetologia 52:42-5.
- Marfella R, Rossi F, Giugliano D (2001) Hyperglycemia and QT interval: time for re-evaluation. Diabetes Nutr Metab 14:63-5.
- Suys BE, Huybrechts SJ, De Wolf D, Op De Beeck L, Matthys D, Van Overmeire B, Du Caju MV, Rooman RP (2002) QTc interval prolongation and QTc dispersion in children and adolescents with type 1 diabetes. J Pediatr 141:59-63.
- Brown DW, Giles WH, Greenlund KJ, Valdez R, Croft JB (2001) Impaired fasting glucose, diabetes mellitus, and cardiovascular disease risk factors are associated with prolonged QTc duration. Results from the Third National Health and Nutrition Examination Survey. J Cardiovasc Risk 8:227-33.
- Veglio M, Bruno G, Borra M, Macchia G, Bargero G, D’Errico N, Pagano GF, Cavallo-Perin P (2002) Prevalence of increased QT interval duration and dispersion in type 2 diabetic patients and its relationship with coronary heart disease: a population-based cohort. J Intern Med 251:317-24.
- Robinson RT, Harris ND, Ireland RH, Lee S, Newman C, Heller SR (2003) Mechanisms of abnormal cardiac repolarization during insulin-induced hypoglycemia. Diabetes 52:1469-74.
- Lo SS, Sutton MS, Leslie RD (1993) Information on type 1 diabetes mellitus and QT interval from identical twins. Am J Cardiol 72:305-9.
- Iacobellis G, Curione M, Di Bona S, Ribaudo MC, Zappaterreno A, Vecci E, Di Mario U, Leonetti F (2005) Effect of acute hyper – insulinemia on ventricular repolarization in uncomplicated obesity. Int J Cardiol 99:161-3.
- Zhang Y, Han H, Wang J, Wang H, Yang B, Wang Z (2003) Impairment of human etherà- go-go-related gene (hERG) K+ channel function by hypoglycemia and hyperglycemia. Similar phenotypes but different mechanisms. J Biol Chem 278:10417-26.
- Du XL, Edelstein D, Rossetti L, Fantus IG, Goldberg H, Ziyadeh F, Wu J, Brownlee M (2000) Hyperglycemia-induced mitochondrial superoxide overproduction activates the hexosamine pathway and induces plasminogen activator inhibitor-1 expression by increasing Sp1 glycosylation. Proc Natl Acad Sci 97:12222-6.
- Nishikawa T, Edelstein D, Du XL, Yamagishi S, Matsumura T, Kaneda Y, Yorek MA, Beebe D, Oates PJ, Hammes HP, Giardino I, Brownlee M (2000) Normalizing mitochondrial superoxide production blocks three pathways of hyperglycaemic damage. Nature 404:787-90.
- Oda A, Bannai C, Yamaoka T, Katori T, Matsushima T, Yamashita K (1994) Inactivation of Cu,Zn-superoxide dismutase by in vitro glycosylation and in erythrocytes of diabetic patients. Horm Metab Res 26:1-4.
- Ytrehus K, Myklebust R, Mjøs OD (1986) Influence of oxygen radicals generated by xanthine oxidase in the isolated perfused rat heart. Cardiovasc Res 20:597-603.
- Patanè G, Anello M, Piro S, Vigneri R, Purrello F, Rabuazzo AM (2002) Role of ATP production and uncoupling protein-2 in the insulin secretory defect induced by chronic exposure to high glucose or free fatty acids and effects of peroxisome proliferator-activated receptor-gamma inhibition. Diabetes 51:2749-56.
- Hreiche R, Plante I, David LP, Simard C, Turgeon J, Drolet B (2009) Impact of glucose concentration on cardiac ventricular repolarization under I Kr /I Ks blocking agents. J Mol Cell Cardiol 47:210-20.
- Watanabe T, Ashikaga T, Nishizaki M, Yamawake N, Arita M (1997) Association of insulin with QTc dispersion. Lancet 350:1821-2.
- Nishizaki M, Ashikaga T, Yamawake N, Fujii H, Arita M, Sumitomo N, Sakurada H, Hiraoka M (2002) Effects of glucose-induced insulin secretion on ventricular repolarization in patients with congenital long QT syndrome. Circ J 66:35-40.
- Nishizaki M, Sakurada H, Ashikaga T, Yamawake N, Fujii H, Arita M, Isobe M, Hiraoka M (2003) Effects of glucose-induced insulin secretion on ST segment elevation in the Brugada syndrome. J Cardiovasc Electro – physiol 14:243-9.
- Hansen PS, Buhagiar KA, Gray DF, Rasmussen HH (2000) Voltage-dependent stimulation of the Na+-K+ pump by insulin in rabbit cardiac myocytes. Am J Physiol Cell Physiol 278:C546-53.
- Aulbach F, Simm A, Maier S, Langenfeld H, Walter U, Kersting U, Kirstein M. (1999) Insulin stimulates the L-type Ca2+ current in rat cardiac myocytes. Cardiovasc Res. 42:113-20.
- Sanguinetti MC, Jurkiewicz NK (1992) Role of external Ca2+ and K+ in gating of cardiac delayed rectifier K+ currents. Pflugers Arch 420:180-6.
- Yang T, Roden DM (1996) Extracellular potassium modulation of drug block of IKr. Implications for torsade de pointes and reverse use-dependence. Circulation 93:407-11.
- Yang T, Snyders DJ, Roden DM. (1997) Rapid inactivation determines the rectification and [K+]o dependence of the rapid component of the delayed rectifier K+ current in cardiac cells. Circ Res 80:782-9.
- Hanton G, Yvon A, Provost JP, Racaud A, Doubovetzky M. (2007) Quantitative relationship between plasma potassium levels and QT interval in beagle dogs. Lab Anim 41:204-17.
- Massaeli H, Guo J, Xu J, Zhang S (2010) Extracellular K+ is a prerequisite for the function and plasma membrane stability of hERG channels. Circ Res 106:1072-82.
- Guo J, Massaeli H, Xu J, Jia Z, Wigle JT, Mesaeli N, Zhang S (2009) Extracellular K+ concentration controls cell surface density of IKr in rabbit hearts and of the HERG channel in human cell lines. J Clin Invest 119:2745-57.
- Cohen JD, Neaton JD, Prineas RJ, Daniels KA (1987) Diuretics, serum potassium and ventricular arrhythmias in the Multiple Risk Factor Intervention Trial. Am J Cardiol 60:548-54.
- Berger E, Patel K, Anwar S, Davies W, Sheridan DJ (2005) Investigation of the effects of physiological and vasodilation-induced autonomic activation on the QTc interval in healthy male subjects. Br J Clin Pharmacol 60: 17-23.
- Shusterman V, Beigel A, Shah SI, Aysin B, Weiss R, Gottipaty VK, Schwartzman D, Anderson KP (1999) Changes in autonomic activity and ventricular repolarization. J Electrophys 32:185-192.
- Wallis RM (2010) Integrated risk assessment and predictive value to humans of non-clinical repolarization assays. Br J Pharmacol 159:115-21.
- Thomas D, Kiehn J, Katus HA, Karle CA (2004) Adrenergic regulation of the rapid component of the cardiac delayed rectifier potassium current, IKr, and the underlying hERG ion channel. Basic Res Cardiol 99:279-87.
- Kurokawa J, Bankston JR, Kaihara A, Chen L, Furukawa T, Kass RS (2009) KCNE variants reveal a critical role of the beta subunit carboxyl terminus in PKA-dependent regulation of the IKs potassium channel. Channels 3:16-24.
- Barros F, Gomez-Varela D, Viloria CG, Palomero T, Giráldez T, de la Peña P (1998) Modulation of human erg K+ channel gating by activation of a G protein-coupled receptor and protein kinase C. J Physiol 511:333-46.
- Cockerill SL, Tobin AB, Torrecilla I, Willars GB, Standen NB, Mitcheson JS (2007) Modulation of hERG potassium currents in HEK-293 cells by protein kinase C. Evidence for direct phosphorylation of pore forming subunits. J Physiol 581:479-93.
- Heath BM, Terrar DA (2000) Protein kinase C enhances the rapidly activating delayed rectifier potassium current, IKr , through a reduction in C-type inactivation in guinea-pig ventricular myocytes. J Physiol 522:391-402.
- Chen J, Chen K, Sroubek J, Wu Z-Y, Thomas D, Bian J-S, McDonald TV (2010) Posttranscriptional control of hERG potassium channel by α adrenergic receptor stimulation Mol Pharm (http://molpharm.aspet journals.org/content/early/2010/05/11/mol.1 09.062216.full.pdf)
- Wittstein IS, Thiemann DR, Lima JA, Baughman KL, Schulman SP, Gerstenblith G, Wu KC, Rade JJ, Bivalacqua TJ, Champion HC (2005) Neurohumoral features of myocardial stunning due to sudden emotional stress. N Engl J Med 352:539-48.
- Smith AH, Norris KJ, Roden DM, Kannankeril PJ (2007) Autonomic tone attenuates drug-induced QT prolongation. J Cardiovasc Electrophysiol 18:960-4.
- Nidhi GM, Davies JW, Jenkins JL (2006) Prediction of biological targets for compounds using multiple-category Bayesian models trained on chemogenomics databases. J Chem Inf Model 46: 1124-1133.
- Keiser MJ, Setola V, Irwin JJ, Laggner C, Abbas AI, Hufeisen SJ, Jensen NH, Kuijer MB, Matos RC, Tran TB, Whaley R, Glennon RA, Hert J, Thomas KL, Edwards DD, Shoichet BK, Roth BL (2009) Predicting new molecular targets for known drugs. Nature 462: 175-181.
- Friberg LE, Isbister GK, Duffull SB (2005) Pharmacokinetic-pharmacodynamic modeling of QT interval prolongation following citalopram overdoses. Br J Clin Pharmacol 61: 177-190.
- Wible BA, Hawryluk P, Ficker E, Kuryshev YA, Kirsch G, Brown AM (2005) HERG-Lite: a novel comprehensive high-throughput screen for drug-induced hERG risk. J Pharmacol Toxicol Methods 52:136-45.
About the Authors
Gül Erdemli
Gül Erdemli obtained an MD from Hacettepe University, Medical School, Ankara, Turkey and a PhD in Pharmacology from the same university. She did her postdoctoral studies at McGill University in Montreal, Institute of Neurology and Neurosurgery in London and Cardiff University until establishing her lab at University of Liverpool as a lecturer. In 2003, she joined Organon Laboratories / Schering Plough Research Institute in UK (now a part of Merck Research Laboratories) as the electrophysiology team leader. In October 2008, she joined Novartis Institutes for BioMedical Research and is the Head of Ion Channel Group in Cambridge, Massachusetts. Her group is responsible for in vitro cardiac ion channel safety profiling and supports ion channel drug discovery projects.
Contact the Author
email: [email protected]
Dmitri Mikhailov
Dmitri Mikhailov holds an MSc in Physics & Applied Math and a PhD in Structural Biology with the focus on using NMR and modelling to study interactions between proteins and natural products. After a post-doctorate at Harvard in 1998-99, Dmitri joined SGI Life Sciences where he was a Bioinformatics Application Scientist responsible for major academic and biopharmaceutical client collaborations. In 2003, Dmitri joined Novartis in Cambridge, US to establish Advanced Scientific Computing team responsible for developing global scientific web tools. Since 2007, Dmitri’s focus has been on safety assessment and using informatics approaches to manage early drug safety risks and to explore drug repositioning opportunities.
Albert Kim
Albert M Kim earned a bachelor’s degree in Biomedical Engineering at Harvard University and attended medical school at UCLA. As a member of the medical scientist training program there, he earned his M.D. and a Ph.D. in Physiology. He then completed an internal medicine residency at Brigham and Women’s Hospital in Boston, a cardiology fellowship at Massachusetts General Hospital in Boston and a cardiac electrophysiology fellowship at UCSF. He joined the UCSF faculty as a clinician-scientist in 2008 where his research focused on new therapies for atrial fibrillation. He was recruited to the Novartis Institutes for Biomedical Research in 2009 where he now serves as a Senior Translational Medicine Expert in cardiovascular medicine and is an active member of the Translational Cardiovascular Advisory Team. He also practices clinical electrophysiology at the West Roxbury VA Hospital.