Controlled nucleation in freeze-drying
Posted: 22 October 2012 |
The stochastic nature of nucleation during the freezing step of the freeze-drying process has been regarded as a demerit in a process which is considered under rigorous control. The freezing performance of a product can impact its subsequent drying behaviour and the final product quality attributes. Hence, the idea to control this stochastic event and thus to directly influence the product morphology seems highly appealing. Sound understanding of the nature of nucleation and its link to drying performance, as well as the choice of a suitable technical concept, is of fundamental importance and the prerequisite to profit from the opportunities offered by controlled nucleation.
Freeze-drying is a commonly used method within the pharmaceutical industry. One of the key steps of the entire process is the initial freezing procedure. During freezing of an aqueous solution, the formation of ice does not start at the equilibrium freezing temperature, Tf (Figure 1). Instead, the solution shows supercooling below Tf until the first ice nuclei are formed at the nucleation temperature, Tn. Nucleation itself proceeds in a three-phase process. ‘Primary nucleation’ describes the point where initial crystal nuclei appear from molecular clusters exceeding a critical size1,2. The formed nuclei are further grown to ice crystals by secondary nucleation (also referred to as ‘crystallisation’) passing through the already nucleated volume1.
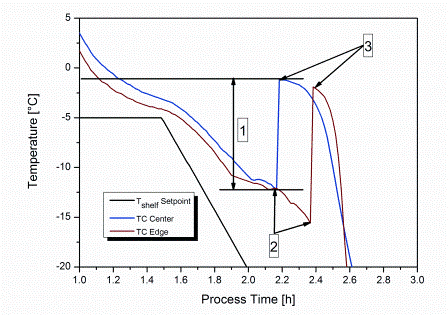
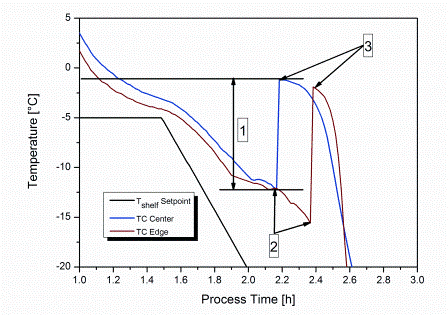
Figure 1: Typical product temperature profiles during uncontrolled nucleation indicated by thermocouples placed centre bottom within the vial. 1: degree of supercooling; 2: nucleation temperature, Tn; 3: equilibrium freezing temperature, Tf.
The exothermal nature of crystallisation leads to an increase of product temperature up to Tf, and thus stops secondary nucleation. In the last phase of nucleation, final solidification proceeds from the shelf-cooled vial bottom towards the top. As the heat of crystallisation must be transferred from the solidification interface through the already-solidified matrix and the vial bottom to the cooled shelf, this step is completed relatively slowly. The temperature difference between Tf and Tn is known as supercooling and was found to be as high as 30°C or more in a GMP manufacturing facility with lowparticulate environment3-5.
The connection between nucleation behaviour and drying performance of the product becomes obvious when considering the size and shape of ice crystals as being the ‘negative templates’ for the pores and cavities of the dried product matrix. Hence, ice morphology decisively determines the resistance to water vapour flow (Rp) from the ice sublimation interface through the already dried product during the drying process. It has been well documented that the extent of supercooling correlates to ice crystal size in an inverse manner. As an example, large ice crystals resulting from a low degree of supercooling impose a lower Rp, and thus elevate the effective primary drying rate6,7. The specific surface area (SSA) in the dried product is lower if larger ice crystals are formed during freezing. An increase of about four per cent in primary drying rate has been reported for every degree Celsius reduction in supercooling1. However, it should also be noted that formulations with lower SSA were found to exhibit reduced moisture desorption rates during secondary drying8. Overall, the above mentioned arguments underline the importance of the freezing step for all subsequent stages of the freezedrying process.
Annealing to improve the ice crystal size distribution in a batch
The nature of ice nucleation is spontaneous and random, and the effective nucleation temperature depends on several aspects. These factors include solution properties, process conditions, environmental influences, characteristics of the container and the presence of particulate matter5. Therefore, a certain level of variability in freezing within a batch and between batches is expected. This difference in freezing behaviour is one of the challenges that needs to be overcome when scaling a cycle from laboratory to manufacturing. Frequently, annealing is used to reduce the differences in ice crystal morphology between vials in a batch. The concept of annealing encompasses the increase in shelf temperature above Tg’ (glass transition temperature of the maximally freeze-concentrated solute) while maintaining the product temperature below the onset of ice melting5,9. The growth of larger ice crystals is promoted at the expense of smaller ones, causing a reduction of heterogeneity in crystal size (‘Ostwald Ripening’). However, annealing requires sufficient time to achieve the desired effect and is also of limited applicability for formulations susceptible to phase separation, buffer crystallisation, interfacial degradation and / or degradation reactions above Tg’5.
Technical concepts to control nucleation
To minimise the impact of freezing variability during the freezing step in a lyophilisation cycle, various concepts have been presented to manipulate or even control nucleation. In general, uncontrolled nucleation occurs over a wide range of product temperatures (typically between -5°C and -15°C in the laboratory) and over a period of time (e.g. 30 – 40 minutes) within a batch. Hence, the term ‘control’ needs to be defined as the ability to assign both time and temperature at which nucleation occurs. The capability of a technical concept for nucleation control should therefore be judged by the ability to provide such ‘two-dimensional control’. An overview of the most relevant technical approaches follows.
Seeding approaches and vial pre-treatment
An early approach which was suggested for nucleation control is the use of additives, such as inorganic compounds (e.g. silver iodid) or bacteria (e.g. pseudomonas syringae)10,11. Further, vial pre-treatment (scoring, scratching or surface roughening) was found to be a way to generate additional nucleation sites and thereby promote ice crystal formation5. However, it is clear that the use of additives or enhancement of surface roughness does not enable control of nucleation in the above-defined sense. Instead, their presence increases the average nucleation temperature. In addition, such techniques are clearly not applicable for manufacture of freeze dried parenteral products based on the respective stringent regulations for sterility and particulate matter4,12.
Electro-freezing and ultrasound nucleation
An additional method proposed by Petersen et al succeeded to induce nucleation by using a strong electric pulse6. This concept, however, also requires an electrode which is in direct contact with the product. Further, the concept cannot be directly applied to solutions containing significant amounts of salts. A few years ago, vibration was described as a way to induce nucleation in a metastable system, which is also referred to as (ultra)sonic-nucleation13-15. The concept uses a short acoustic pulse (approximately 10 metres per second) in a frequency range of 10 – 40 kHz to induce crystal formation. This technology, however, never succeeded in a large scale environment.
Vacuum induced freezing
A further concept to induce nucleation was described as ‘vacuum induced surface freezing’ by Kramer et al16,17 which was further investigated by Liu et al18. In this procedure, surface freezing is induced by decreasing the pressure in the freeze-drying chamber to negative pressure (about one mbar) after the liquid product was equilibrated at a given shelf temperature. The initiation of vacuum prior to solidification promotes the risk of excessive boiling and impairment of product appearance, which has been pointed out as the major drawback of this procedure4.
Ice fog technique
The use of an ice fog was first suggested by Rowe19 and described for freeze-drying processes by Rhambhatla et al in 20047. The basic concept is the introduction of cold nitrogen gas at atmospheric or moderate negative pressures into the product chamber to freeze out moisture within the chamber atmosphere5,7. The small ice crystals within the formed ice fog induce nucleation at the surface of the product solution. The density of the formed ice fog and its distribution in the chamber must be sufficiently high to reliably ensure nucleation in all vials. Most recent ice fog concepts utilise a steam generator and a heat exchanger to allow the formation of such dense and uniform ice fog, even in manufacturing scale equipment20. Further implementations of this technique manage to generate an ice fog in the condenser by utilising moisture and the pre-cooled condenser coils. Subsequently, the formed ice fog is ‘sucked’ into the partially evacuated product chamber to induce nucleation.
Depressurisation
A new method based on the concept of pressure variation was described in a patent application by Gasteyer et al in 200721. Here, the product solution was first brought to a temperature slightly below its Tf. In a next step, the freezedrying chamber was pressurised with an inert gas such as argon to about 28 psig (2.94 bar)4,21. To induce nucleation the overpressure was released within 10 seconds or less (Figure 2). For 100 per cent nucleation in a typical freeze-drying setup, a pressure change of at least 7 psi (=^ approximately 0.5 bar, argon) was suggested21. Once nucleation has been induced, the shelf temperature is either immediately reduced (Figure 2) to shorten the time required to fully solidify the product or maintained at the shelf setpoint for nucleation control for an additional period of time to further grow the ice crystals.
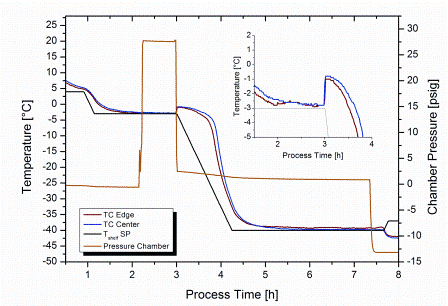
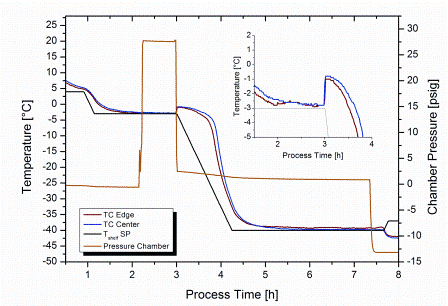
Figure 2: Overview of product temperature and chamber pressure during nucleation control by depressurisation from 28 psig (2.94 bar) to 2 psig (1.15 bar). Nucleation temperature setpoint: -3°C. Insertation provides a magnification of instantaneous temperature increase due to crystallisation in the moment of pressure release
Different mechanisms have been discussed as inducing factors for crystal formation pertinent to this method, or at least partially contributing to the achieved effect: first, the decrease in gas solubility in the solution upon pressure release, resulting in gas bubble formation21. Secondly, evaporation and corresponding endothermic cooling at the liquid surface caused by the sudden pressure decrease, which could induce surface freezing21. Thirdly, the depressurised gas in proximity to the solution surface is cooled down adiabatically and could also freeze water vapour, either present in equilibrium with the solution prior to pressure release or liberated from the product by evaporation during depressurisation21. The ice crystals (frozen water vapour) would act as seeds and thus induce nucleation. Although the mechanism of nucleation for this procedure is not yet fully understood, one important observation has been already made: freezing in a vial progresses from top to bottom of the solution when using this procedure4. This is in direct contrast to traditional shelf freezing.
Note that freeze dryers used to execute such nucleation control must align with some basic technical requirements. While the capability to establish and withstand the necessary over pressurisation could be easily achieved in systems comprising CIP/SIP, removal of the gas introduced during the chamber pressurisation within the required (very short) timeframe can be a far more challenging undertaking, especially on large scale equipment.
A laboratory case study: impact of nucleation control on drying performance
A set of laboratory experiments were conducted to evaluate the influence of different freezing regimes (including random shelf freezing, annealing and controlled nucleation) on drying performance. A 75 mg/mL sucrose model formulation (246 vials, 5 mL, 2.5 mL fill volume) was freeze-dried in a laboratory 0.46 m2 freezedryer equipped with SMARTTM and a nucleation on-demand technology to control nucleation by depressurisation4,21. Product temperature, Tp-TC, was monitored by using thermocouples (36 gauge) attached to the outside of the vials. As a reference cycle, the product was conventionally frozen using a slow shelf cooling rate (0.5°C/minute). The primary drying step was optimised by applying SMARTTM Freeze Dryer technology22. Note that SMARTTM is a process analytical technology tool which provides product interface temperature (Tp-MTM) and resistance (Rp) data for the batch as a whole23. The evaluated optimum primary drying conditions were then analysed in combination with the different freezing procedures, and the resulting product performance was directly compared.
Product performance during primary drying
As expected, the product morphology formed by uncontrolled nucleation (Tn between -11°C and -16°C) imposed the highest Rp (Figure 3) and, in turn, the highest average Tp-TC during primary drying. In contrast, controlled nucleation at -3°C (i.e. slightly below Tf of the solution) clearly promoted the formation of larger ice crystals. As a consequence, elevated mass flow rates during primary drying were obtained which could be confirmed by the approximately 1°C lower SMARTTM product temperature, Tp-MTM. In addition, Rp was about 25 per cent reduced, compared to random nucleation (Figure 3).
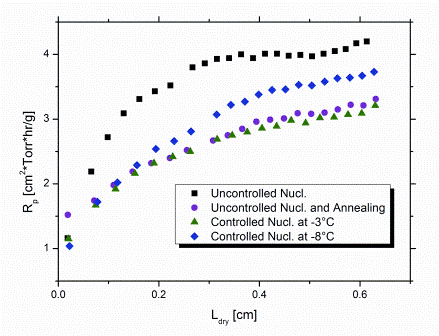
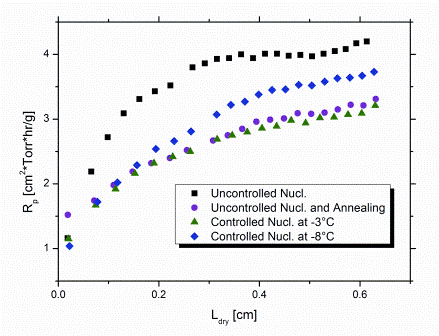
Figure 3: Overview of product resistance profiles calculated from MTM pressure rise data (SMARTTM) for different freezing procedures
Control of Tn at a higher degree of supercooling (at -8°C) also led to a reduction in Rp. The reason for this is the still lower extent of supercooling compared to uncontrolled freezing. In addition, the occurance of nucleation in all vials at identical time and temperature results in a high degree of batch uniformity with regard to product morphology. This contributes to a reduction in Rp as well. Note that Rp data determined by SMARTTM always represent an average value for the entire batch.23 Application of an annealing step after uncontrolled freezing (0.5°C/minute cooling rate to -40°C, hold for 120 minutes; subsequent annealing: -15°C over six hours) reduced Rp by about the same extent as controlled nucleation at -3°C. Corresponding reduction of primary drying time, however, was less pronounced (10 per cent versus 15 per cent at -3°C; Figure 4) and only comparable to the effect achieved by control of Tn at -8°C. This might suggest that subsequent implementation of an annealing step for products originally formed by uncontrolled nucleation ultimately results in a lower degree of overall batch homogeneity, relative to controlled nucleation. With the degree of supercooling and, in turn, the distribution of nucleation temperatures being even more pronounced in sterile manufacturing, the benefit in efficiency for controlled nucleation is expected to become even more significant on production scale. In addition, the required freezing step time for controlled nucleation is clearly shorter than for annealing. In the present study, comparable savings in primary drying time (approximately 10 per cent) were achieved by controlled nucleation at -8°C and annealing. However, the overall shortening of the total cycle for the same cycles was about 18 per cent higher for controlled nucleation, because the time of the freezing step was only half for the controlled nucleation procedure. In these laboratory experiments, a maximum saving in cycle time of approximately six per cent could be achieved when controlling Tn at -3°C compared to random nucleation.
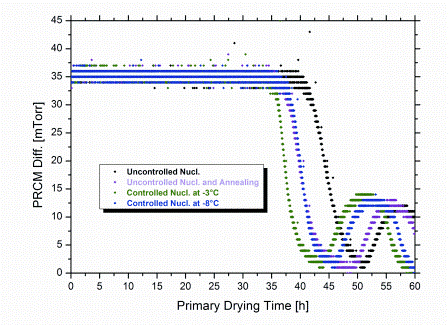
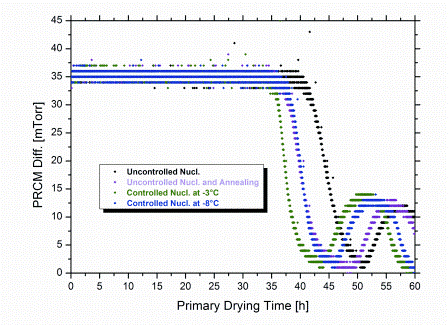
Figure 4: Pressure difference between Pirani sensor and Capacitance Manometer reading (PRCM Diff.) during primary drying. For determination of primary drying times an endpoint criterion of PRCM Diff. = 2 mTorr (ca. 3 μbar) was used
Secondary drying performance and residual moisture
Since identical secondary drying conditions were used in all experiments (heating rate: 0.1°C/minute to +40°C, six hours secondary drying time), the final residual moisture (RM) content well reflected the suggested decrease in moisture desorption rate during secondary drying for products with increased crystal size and, in turn, reduced SSA8. In comparison to uncontrolled frozen product (RM about 0.2 per cent), the moisture content of product prepared using controlled nucleation at -3°C and -8°C showed elevated values (RM: 0.6 per cent and 0.35 per cent respectively). This observation suggests that secondary drying efficiency may be compromised when using the same conditions as for application of a conventional freezing procedure. In turn, it demonstrates the need for adjustment of the secondary drying step if product is frozen under control of Tn.
Cake appearance and inner product morphology
Product microstructure analysed by SEM (scanning electron microscopy) confirmed the increase in ice crystal size and corresponding pore size (Figure 5, page 66). The macroscopic cake elegancy was overall acceptable but revealed a more porous and fragile structure for the cakes prepared using controlled nucleation. Less efficient compensation of distortion by global shrinkage due to minor mechanical strength of the product matrix most likely forms the basis for enhanced cracking within such cakes. Application of a vial system equipped with surface coating to reduce adhesive forces between product and vial wall could be a valuable approach to improve this potential compromise.
Conclusions
The freezing step in a freeze-drying process must be considered as more than just a starting point and fundamental requirement to remove moisture by sublimation. The freezing step is the key to enhanced process and product optimisation, and control of nucleation as the main principle to achieve this goal. Moreover, control of Tn is a useful method to increase product uniformity and facilitate cycle transfer and scale-up procedures. ‘True’ control of this naturally random event must be understood as the ability to assign both time and temperature of ice crystal formation. Several technical concepts have been proposed to fulfill this task, but only few of them are potentially applicable within the environment of large scale pharmaceutical freeze-drying. Finally, laboratory data suggest the strategy of controlled nucleation to be not only in theory in a promising concept with regard to cycle optimisation and drying performance. Compared to the proven concept of annealing, the merit of this approach is founded on shorter freezing step times required and the high degree of uniformity achieved by simultaneous nucleation in all product containers. If secondary drying conditions are adapted to the requirements of the changed product morphology, the control of nucleation temperature enables optimisation of the freeze-drying process beyond the boundaries dictated by a stochastically formed product microstructure.
References
1. J. A. Searles, J. F. Carpenter, T. W. Randolph. The Ice Nucleation Temperature Determines the Primary Drying Rate of Lyophilization for Samples Frozen on a Temperature-Controlled Shelf. J. Pharm. Sci. (2001) 90(7): 860-871
2. D. Kashchiev, G. M. van Rosmalen. Review: Nucleation in Solutions Revisited. Crys. Res. Technol. (2003) 7(8): 555-574
3. X. Tang, M. J. Pikal. Design of Freeze-Drying Processes for Pharmaceuticals: Practical Advice. Phram. Res. 21(2): 191-200
4. A. K. Konstantinidis, W. Kuu, L. Otten, S. L. Nail, R. R. Sever. Controlled Nucleation in Freeze-Drying: Effects on Pore Size in the Dried Product Layer, Mass Transfer Resistance, and Primary Drying Rate. J. Pharm. Sci. (2011) DOI 10.1002/jps.22561
5. S. M. Patel, C. Bhugra, M. J. Pikal. Reduced Pressure Ice Fog Technique for Controlled Ice Nucleation during Freeze-Drying. AAPS Pharm. Sci. Tech. (2009) 10(4): 1406-1411
6. S. Petersen, G. Rau, B. Glasmacher. Reduction of Primary Freeze-Drying Time by Electric Field Induced Ice Nucleus Formation. Heat Mass Transfer (2006) 42(10): 929-938
7. S. Rhambhatla, R. Ramot, C. Bhugra, M. J. Pikal. Heat and Mass Transfer Scale-up Issues during Freeze- Drying: II. Control and Characterization of the Degree of Supercooling. AAPS Pharm. Sci. Tech. (2004) 5(4): Article 58
8. M. J. Pikal, S. Shah, M. L. Roy, R. Putman. The Secondary Drying Stage of Freeze-Drying: Drying Kinetics as a Function of Temperature and Chamber Pressure. Int. J. Pharm. (1990) 60(3): 203-217
9. J. A. Searles, J. F. Carpenter, T.W. Randolph. Annealing to Optimize the Primary Drying Rate, Reduce Freezing- Induced Drying Rate Heterogeneity, and Determine Tg’ in Pharmaceutical Lyophilization. J. Pharm. Sci. (2001) 90(7): 972-887
10. P. Widehem, N. Cochet. Pseudomonas Syringae as an Ice Nucleator – Application to Freeze-Concentration. Process Bio-chem. (2003) 39(4):405-410
11. K. E. Zachariassen, E. Kristtiansen. Ice Nucleation and Antinucleation in Nature. Cryobiology (2000) 41(4): 257-279
12. A. Petersen, H. Schneider, G. Rau, B. Glasmacher. A New Approach for Freezing of Aquaous Solutions Under Active Control of the Nucleation Temperature. Cryobiology (2006) 53(2): 248-257
13. A. Hottot, K. Nakagawa, J. Andrieu. Effect of Ultrasound Controlled Nucleation on Structural and Morphological Properties of Freeze-Dried Mannitol Solutions. Chem. Eng. Res. Des. 86(2): 193-200
14. K. Nakagawa, A. Hottot, S. Vessot, J. Andrieu. Influence of Controlled Nucleation by Ultrasound on Ice Morphology of Frozen Formulations for Pharmaceutical Proteins Freeze-Drying. Chem. Eng. Process. (2006) 45(9):783-791
15. M. Scalier, R. Peczalski, J. Andrieu. Effect of Ultrasonically Induced Nucleation on Ice Crystals’ Size and Shape During Freezing in Vials. Chem. Eng. Sci. (2010) 65(10): 3064-3071
16. M. Kramer, B. Sennhenn, G. Lee. Freeze-Drying Using Vacuum-Induced Surface Freezing. J. Pharm. Sci. (2002) 91(2):433-443
17. B. Sennhenn, M. Kramer. Lyophilizaion Method. (2004). Patent US6.684.524
18. J. Liu. T. Vivrette, M. Virgin, M. Anderson, P. Dalal. A Study of the Impact of Freezing on the Lyophilization of a Concentrated Formulation with a High Fill Depth. Pharm. Dev. Tech. (2005) 10(2):261-272
19. T. W. Rowe. A Technique for the Nucleation of Ice. International Symposium on Biological Product Freeze-Drying and Formulation. Switzerland: Geneva; 1990
20. P. Chakravarty, R. Lee, F. DeMarco, E. Renzi. Ice Fog as a Means to Induce Uniform Ice Nucleation During Lyophilization. Bio. Pharm. Int. (2012) 25(1): 33-38
21. T. H. Gasteyer, R. R. Sever, B. Hunek, N. Grinter, M. L. Verdone. Lyophilization System and Method. (2007). Patent US20070186437
22. X. C. Tang, S. L. Nail, M. J. Pikal. Evaluation of Manometric Temperature Measurement (MTM), a Process Analytical Technology Tool in Freeze-Drying, Part III: Heat and Mass Transfer Measurement. AAPS Pharm. Sci. Tech. (2006) 7(4): Article 97
23. X. C. Tang, S. L. Nail, M. J. Pikal. Evaluation of Manometric Temperature Measurement, a Process Analytical Technology Tool for Freeze-drying: Part II Measurement of Dry-layer Resistance. AAPS Pharm. Sci. Tech. (2006) 7(4): Article 93
About the authors
Peter Stärtzel is a licensed pharmacist in Germany and currently employed as a pharmaceutical scientist at GILYOS GmbH in Wuerzburg, Germany. At GILYOS, he also pursues his PhD in pharmaceutics under direct supervision of Dr. Henning Gieseler.
Dr. Henning Gieseler has been a licensed pharmacist in Germany since 2000 and received his PhD at the Department of Pharmaceutics, University of Erlangen (Germany) in 2004. After a post-doctoral research period at the School of Pharmacy, University of Connecticut (Professor Michael J. Pikal) until early 2006, he returned as an Assistant Professor to the University of Erlangen where he founded the ‘Freeze Drying Focus Group’ (FDFG) within the Division of Pharmaceutics. In 2010, Dr. Gieseler obtained his habilitation and venia legendi in Pharmaceutical Sciences from the University of Erlangen with the thesis ‘Quality by Design (QbD) in Freeze-Drying Using Advanced Process Analytical Technology (PAT)’. In the same year, he formed GILYOS GmbH (www.gilyos.com), a contract freeze-drying service provider for the pharmaceutical and food industry. Dr. Gieseler currently holds a position as CEO at GILYOS and as an Associate Professor at the Division of Pharmaceutics, University of Erlangen.