A history of recombinant protein technology in small molecule drug discovery
Posted: 28 October 2014 | | No comments yet
Recombinant protein production is a prerequisite and essential component of most modern small molecule drug discovery programs. Target proteins are required to underpin screening, structural and mechanistic studies providing data that drives chemical design. From the initial establishment of recombinant protein production in the pharmaceutical industry in the 1980s, systems and technologies have evolved in step with developments in other areas to enable rapid production of many different target proteins, and their variants, specifically designed for their end use. This review describes the evolution of recombinant protein production over the past 30 years, tracking changes in technologies and working practices in relation to landmark changes in drug discovery strategies over that period.
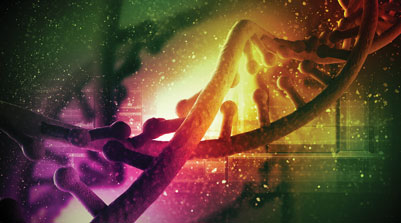
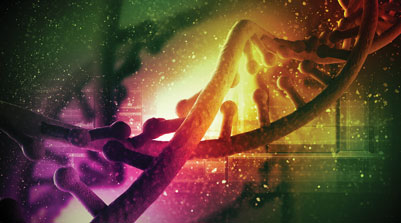
Rise of the recombinant protein expression systems
Throughout the 1980s the development of key technologies enabling recombinant protein production progressed rapidly. However, in the pharmaceutical industry, protein targets were still largely extracted and purified from animal tissue. Consequently, many projects were driven by data that was derived using proteins from non-human species and the throughput of many assays was limited by the amount of protein which could be purified. Many batches of protein were typically required to underpin the project, and batch-to-batch variability was a frequent issue. The situation changed in the early ‘80s with the establishment and commercialisation of automated Edman sequencers1. This new technology provided the opportunity to generate amino acid sequences from proteins previously isolated by classical biochemical purification from animal tissue sources. Sequence information could then be used to design probes to allow cloning of human genes. This was a labour-intensive process but did enable the generation of human genes which could be inserted into an ever increasing set of emerging vectors for different recombinant expression systems.
Following the first recombinant expression in Escherichia coli, which took place in 1976 when scientists at Genentech – the first genetic engineering company – produced the human protein Somatostatin2, protein production systems that used bacteria continued to improve. Eukaryotic protein expression systems started to be developed, including the insect cell system that utilised baculovirus3 and the Pichia pastoris4 yeast expression system, providing the opportunity for more complex, glycosylated protein production.
Access to commercial molecular cloning reagents, coupled with the increasingly widespread usage of polymerase chain reaction (PCR)5 for gene amplification during the 1990s further accelerated the uptake and implementation of these expression systems by the pharmaceutical industry. One of the key breakthroughs of the early ‘90s was the development of the T7 polymerase lac repressor-based pET vector system for E. coli expression6, which provided the platform for bacterial expression to become the standard first-line workhorse for protein production.
Bacterial expression was not only important in supporting biochemical assay generation and X-ray crystallography but also for the emerging field of protein nuclear magnetic resonance (NMR) spectroscopy for structure determination7 and ligand screening, both of which require isotopically labelled proteins. The development of media methods for E. coli growth using controlled sources of nitrogen and carbon, as well as the ability to grow in deuterium, were instrumental in enabling this methodology8. However, the technology has been frequently hampered by low expression yields and the requirement for large culture volumes to produce the required amounts of protein.
Further developments during the ‘90s led to the use of specifically labelled samples produced using autotrophic strains of E. coli to aid resonance assignment, which reduced the requirement for deuturation9. Growth of P. pastoris in minimal media was also possible but was not universally successful and was not adopted for routine production of labelled protein. Towards the end of ‘90s, the development of defined rich media enabled the production of isotopically labelled protein at higher yields for NMR structural studies.
Following a period of successful utilisation of the bacterial expression systems, it became apparent that for more complex proteins the high expression rate in bacteria often exceeded the capacity of the bacterial folding machinery to produce fully functional folded protein, resulting in the formation of inclusion bodies. Standard strategies, including temperature reduction and induction condition manipulation, were – and continue to be – employed to slow down the expression rate alongside newer strategies such as co-expression in the presence of chaperone complexes10.
The development of the Bac-to-Bac system in 199711 for use in insect cell expression allowed for the efficient large-scale production of recombinant baculovirus containing human gene inserts, which were becoming more readily accessible from commercially-available cDNA libraries. In our own labs the insect cell system became a second-line expression system for those intracellular proteins that failed to express at sufficient levels in E. coli (Figure 1). However, although the insect system proved very successful in the production of soluble, functional protein, it was often limited by the level of expression that could be achieved; its primary use being to supply biochemical screening. An alternative to protein expression in higher eukaryotic cells involved mammalian cell-line generation using systems such as the Celltech/Lonza Glutamine Synthetase system (GS)12. This system was well established in the ‘90s but was labour intensive and time consuming, often extending the starting time of discovery programs.
Human genome cracked
The turn of the century saw the much-awaited publication of the human genome sequence13-14, which led rapidly to the identification of many previously unidentified protein sequences. Fast-forward to the current day, and researchers have now drawn up a draft map of the total human proteome15-16, which will be invaluable in understanding the expression patterns of disease-relevant forms of potential target proteins. Concurrent to the human genome project, and its publication, developments in DNA manipulation led to the introduction of more efficient cloning strategies using viral recombinases17 and ligation-independent cloning18. These developments significantly reduced the timelines for expression vector generation, where a single gene could be cloned rapidly into vectors for multiple expression systems.
Access to large quantities of high quality expression vector DNA is typically required for transient transfection of episomally replicating plasmids in HEK293 and CHO (chinese hamster ovary) mammalian cell systems. This process was introduced at a variety of scales throughout the noughties and became the system of choice for secreted proteins, with high levels of expression retaining functionality and solubility19-20. The present decade has seen an increasing shift to expression in mammalian systems and we believe that this trend will continue as the industry continues to explore emerging target classes and protein complexes.
In terms of commercial developments, Gene Synthesis companies began to emerge from the end of the nineties and into the noughties and it quickly became more cost-effective for pharma companies to outsource the generation of expression vectors for the majority of their target proteins, moving away from traditional in-house PCR-based cloning. We have found the advent of synthetic gene methodologies, including library generation, essential in accelerating the identification of soluble, well expressed target protein variants for applications such as crystallography21. In order to facilitate expression trials of multiple constructs, considerable progress was made in the miniaturisation of expression studies22-24. Plate-based expression of multiple constructs was established for E. coli, insect cells, P. pastoris and mammalian cells (CHO and HEK293) in our labs in the noughties, building on developments made in the emerging Structural Genomics Initiatives25 which were working hard to establish processes for the expression of multiple proteins across the genome.
The field of cell-free protein synthesis is also worthy of a mention when discussing small-scale protein synthesis and expression screening. Although we have evaluated a number of these technologies over the past 15 years, we have yet to find a suitable system which satisfies a specific need within our current processes. However, given the considerable advances in this technology – which have enabled cost-effective microscale-to-manufacturing-scale production of complex protein examples26, it is an area which warrants further exploration.
Purification Strategies
In the ‘80s, purification methods for proteins largely relied on separation techniques utilising the inherent physical properties of the target together with more esoteric affinity techniques such as those involving chemical dyes. Development of methods was largely empirical when purifying from tissue sources as the sequence of the protein was unknown. Manual column chromatography began to be superseded by programmable systems (for example, fast protein liquid chromatography (FPLC), G.E. Healthcare) specifically designed to handle proteins. With the emergence of recombinant expression in the industry in the ‘90s, not only did yields of protein increase but more rational approaches to purification based on predicted properties from the sequence (e.g. predicted pI) became more common. The ability to manipulate genes enabled the introduction of tags which could be inserted upstream or downstream of the target sequence. The first reports of MBP27, GST28, Flag29, and 6His30 tags for single-step affinity purification were published in 1988 and provided the opportunity to standardise purification procedures and reduce timelines. However, concerns over the effects of non-native sequences on protein behaviour and properties meant that recombinant proteins were still produced largely untagged and purified by conventional chromatography until the ‘90s. This view changed with the discovery of viral proteases with high specificity for discrete non-human sequences31. These proteases allowed subsequent cleavage of tags and provided the opportunity to produce proteins with an almost completely native sequence. However, problems with the production of Tobacco etch virus (TEV) protease in particular and the relatively high cost limited their use. The development of variants with improved stability and solubility during the noughties meant that TEV protease emerged as the preferred protease and is now regularly used in our labs. Alternative tags developed in the nineties such as Strep-tagII32 and Avi tag (www.Avidity.com) are particularly suitable for the immobilisation of proteins for use in biophysical studies. This became increasingly important as higher throughput and more sensitive surface plasmon resonance (SPR) and other label-free binding technologies were developed in the noughties33. The ability to immobilise targets in a specific orientation using tag systems such as these has enabled screening for weakly binding fragments at high throughput.
Alongside the emergence of recombinant protein expression, the sophistication of chromatography instruments and purification media available for protein purification increased at a similar rate. The introduction of the ӒKTA platform (G.E. Healthcare), BioCad (Perceptive Biosystems) and others allowed for ever more in-depth investigation into the isolation and purification of the target protein from impurities in the ‘90s. In more recent times, the ӒKTAxpress34 and PhyTip (www.phynexus.com) platforms specifically addressed the increased throughput requirement that came with the multiple recombinant protein constructs now routinely designed and expressed. Both systems are being used effectively at either end of the purification scale in our labs in combination with higher resolution affinity resins and traditional chromatography media in pre-packed form. This enables multiple constructs per target to be purified to homogeneity, providing more options for assessment in various applications.
With the last native purification in our labs having been undertaken in 2003 to produce dog Glycogen Phosphorylase from liver tissue, the combination of the 6His tag with TEV protease tag removal and a gel filtration polishing step has become our default purification workhorse. Additional or alternative tags and chromatography steps are introduced as required to increase purity and sample homogeneity, for example, in ion exchange for separating kinase phosphoforms.
Protein analysis and quality control
The purpose for which proteins are generated has always dictated the analytical methods used to assess their quality. In the ‘80s, extracted and purified proteins were tracked through measuring increases in biochemical activity with respect to the total protein concentration, as measured by standard methods such as the Bradford protein assay35. Sodium dodecyl sulfate-polyacrylamide gel electrophoresis (SDS-PAGE) is still used routinely in our labs to track target protein purity throughout the course of purification, although we have evaluated more recent lab-on-a-chip and microfluidic-based approaches. In the absence of a robust biochemical assay, Circular Dichroism can be used to detect secondary structure within the protein and also to determine protein stability36, but is rarely used as a routine analysis technique in our labs. Automated N-terminal sequence analysis1 was used routinely in the late ‘80s to identify initially unknown proteins and eventually protein of known sequence to confirm identity. Low throughput mass spectrometry was available to measure intact mass in the 80s and 90s, but was reserved for analysis of final products. Mass Spectrometry has now become a key analytical tool that allows our group to identify post-translational modifications in our purified proteins (for example, phosphorylation and glycosylation) both generally and more specifically with the use of peptide mapping37. Increased requirements for isotopically labelled protein to support structure activity relationship (SAR) campaigns using NMR fragment screening necessitated accurate mass determination to measure isotope incorporation levels. Native Mass Spectrometry, which allows determination of the mass of protein in a folded state, has allowed measurement of the mass of protein complexes and protein ligand interactions such as those between co-factors and enzymes38. Isothermal titration calorimetry (ITC) became available in the ‘90s and recent advances in miniaturisation and automation have meant that it is more amenable as a routine technique to assess functional purity where a suitable reference ligand is available39.
In the ‘90s, crystallisation of proteins was often a bottleneck, and surrogate analytical endpoints were sought to predict crystallisability of proteins under different formulation conditions. Dynamic Light Scattering (DLS)40 was used to assess homogeneity of samples, but the correlation of DLS data with ultimate propensity to crystallise proved to be variable and the technique has little use in screening constructs for crystallisation now. Moreover the miniaturisation of crystallography screens in the noughties with reduced sample requirements meant that it was no longer necessary to determine surrogate endpoints for crystallisation but to use crystallisation itself as an analytical method to assess protein quality. This has enabled screening of multiple constructs and conditions in an automated fashion41.
Analytical size-exclusion chromatography was routinely used from the nineties and still represents the best way of determining aggregation within samples. Additional tools have been added in more recent years to characterise membrane proteins after solubilisation screens, such as fluorescence-detection size-exclusion chromatography
(FSEC)42, whilst size-exclusion chromatography combined with multi-angle laser light scattering (SEC MALS) is used routinely to assess aggregation in soluble proteins43.
As previously mentioned, concentration measurements were often calculated in the ‘80s and ‘90s using cuvette-based colorimetric assays. As sequence information became available for recombinant proteins, concentration was increasingly determined using absorbance measurements and predicted extinction coefficients. Our group has moved entirely from cuvette-based measurements to low-volume absorbance technologies such as NanoDrop (Thermo Scientific)44.
Innovations in robotics during the late ‘90s and early noughties resulted in the miniaturisation of assays and biophysical methods. The reduction in quantities of protein needed for screens of various sorts (Table 1) necessitated, in the late noughties, the need to miniaturise and parallelise analytical methods. Improvements in front end robotics mean that all the major analytical methods used such as size-exclusion chromatography (SEC), mass spectrometry and SDS-PAGE can now be automated to match demand.
With increased technological advances, the ability to investigate the interactions of proteins, both between themselves and with small molecules, is driving new applications for purified proteins. Direct measurement of ligand interactions is becoming increasingly important as we move into new target areas with no reference compounds. Affinity-based technologies such as Thermofluor are routinely used throughout the protein science community to rapidly identify tool ligands and stabilising agents for use in developing both biophysical and biochemical assays, and protein crystallization45.
Conclusions and future perspectives
Recombinant protein production can now be considered as a mature discipline. This is the result of numerous incremental advances in expression, purification and analysis. It is now rare for a drug discovery program to be stalled as a result of an inability to produce a target protein. Parallel processes for expression, purification and analysis of multiple recombinant proteins are now well established in our labs and across the industry (Figure 2). So what developments might be in store for protein science over the next 30 years? The continued emergence of new phenotypic screening strategies and the greater emphasis on the linkage of targets to disease, rather than a focus on target classes, could demand the production of more complex proteins and diverse protein classes in the future. Protein complexes and the increasing success in generating solubilised membrane proteins will necessitate more changes to keep pace with demand. The emergence of effective contract research organisations, for more routine protein supply, will enable a realignment of protein science resource to tackle these new challenges. Additionally, miniaturisation and parallel workflows should enable the production of complete libraries of proteins (such as the secretome) to enable new target identification and enhanced screening activities, together with the traditional small molecule drug discovery projects. It is our belief that recombinant proteins will continue to be essential reagents for drug discovery projects in the future and that technological developments will, as they have already shown to, emerge to match changes in demand or to keep in step with advances in other application areas.
References
- Niall HD. Automated edman degradation: The protein sequenator. Methods Enzymol. 1973;27:942-1010.
- Itakura K, Hirose T, Crea R, Riggs AD, Heyneker HL, Bolivar F, et al. Expression in escherichia coli of a chemically synthesized gene for the hormone somatostatin. Science. 1977 Dec 9;198(4321):1056-63.
- Smith GE, Summers MD, Fraser MJ. Production of human beta interferon in insect cells infected with a baculovirus expression vector. Mol Cell Biol. 1983 Dec;3(12):2156-65.
- Cregg JM, Vedvick TS, Raschke WC. Recent advances in the expression of foreign genes in pichia pastoris. Biotechnology (N Y). 1993 Aug;11(8):905-10.
- Saiki RK, Gelfand DH, Stoffel S, Scharf SJ, Higuchi R, Horn GT, et al. Primer-directed enzymatic amplification of DNA with a thermostable DNA polymerase. Science. 1988 Jan 29;239(4839):487-91.
- Dubendorff JW, Studier FW. Controlling basal expression in an inducible T7 expression system by blocking the target T7 promoter with lac repressor. J Mol Biol. 1991 May 5;219(1):45-59.
- Wuthrich K. Protein structure determination in solution by NMR spectroscopy. J Biol Chem. 1990 Dec 25;265(36):22059-62.
- Bax A, Ikura M. An efficient 3D NMR technique for correlating the proton and 15N backbone amide resonances with the alpha-carbon of the preceding residue in uniformly 15N/13C enriched proteins. J Biomol NMR. 1991 May;1(1):99-104.
- Waugh DS. Genetic tools for selective labeling of proteins with alpha-15N-amino acids. J Biomol NMR. 1996 Sep;8(2):184-92.
- Ferrer M, Chernikova TN, Yakimov MM, Golyshin PN, Timmis KN. Chaperonins govern growth of escherichia coli at low temperatures. Nat Biotechnol. 2003 Nov;21(11):1266-7.
- Ciccarone VC, Polayes DA, Luckow VA. Generation of recombinant baculovirus DNA in E.coli using a baculovirus shuttle vector. Methods Mol Med. 1998;13:213-35.
- Cockett MI, Bebbington CR, Yarranton GT. High level expression of tissue inhibitor of metalloproteinases in Chinese hamster ovary cells using glutamine synthetase gene amplification. Nat Biotech. 1990 print;8(7):662-7.
- Lander ES, Linton LM, Birren B, Nusbaum C, Zody MC, Baldwin J, et al. Initial sequencing and analysis of the human genome. Nature. 2001 Feb 15;409(6822):860-921.
- Venter JC, Adams MD, Myers EW, Li PW, Mural RJ, Sutton GG, et al. The sequence of the human genome. Science. 2001 Feb 16;291(5507):1304-51.
- Kim MS, Pinto SM, Getnet D, Nirujogi RS, Manda SS, Chaerkady R, et al. A draft map of the human proteome. Nature. 2014 May 29;509(7502):575-81.
- Wilhelm M, Schlegl J, Hahne H, Moghaddas Gholami A, Lieberenz M, Savitski MM, et al. Mass-spectrometry-based draft of the human proteome. Nature. 2014 May 29;509(7502):582-7.
- Hartley JL, Temple GF, Brasch MA. DNA cloning using in vitro site-specific recombination. Genome Res. 2000 Nov;10(11):1788-95.
- Dieckman L, Gu M, Stols L, Donnelly MI, Collart FR. High throughput methods for gene cloning and expression. Protein Expr Purif. 2002 Jun;25(1):1-7.
- Durocher Y, Perret S, Kamen A. High-level and high-throughput recombinant protein production by transient transfection of suspension-growing human 293-EBNA1 cells. Nucleic Acids Res. 2002 Jan 15;30(2):E9.
- Rajendra Y, Kiseljak D, Baldi L, Hacker DL, Wurm FM. A simple high-yielding process for transient gene expression in CHO cells. J Biotechnol. 2011 Apr 20;153(1-2):22-6.
- Overman RC, Green I, Truman CM, Read JA, Embrey KJ, McAlister MS, et al. Stability and solubility engineering of the EphB4 tyrosine kinase catalytic domain using a rationally designed synthetic library. Protein Eng Des Sel. 2013 Oct;26(10):695-704.
- Chambers SP, Austen DA, Fulghum JR, Kim WM. High-throughput screening for soluble recombinant expressed kinases in escherichia coli and insect cells. Protein Expr Purif. 2004 Jul;36(1):40-7.
- McCall EJ, Danielsson A, Hardern IM, Dartsch C, Hicks R, Wahlberg JM, et al. Improvements to the throughput of recombinant protein expression in the baculovirus/insect cell system. Protein Expr Purif. 2005 Jul;42(1):29-36.
- Boettner M, Prinz B, Holz C, Stahl U, Lang C. High-throughput screening for expression of heterologous proteins in the yeast pichia pastoris. J Biotechnol. 2002 Oct 9;99(1):51-62.
- Stevens RC, Yokoyama S, Wilson IA. Global efforts in structural genomics. Science. 2001 Oct 5;294(5540):89-92.
- Carlson ED, Gan R, Hodgman CE, Jewett MC. Cell-free protein synthesis: Applications come of age. Biotechnol Adv. 2012 Sep-Oct;30(5):1185-94.
- Maina CV, Riggs PD, Grandea AG,3rd, Slatko BE, Moran LS, Tagliamonte JA, et al. An escherichia coli vector to express and purify foreign proteins by fusion to and separation from maltose-binding protein. Gene. 1988 Dec 30;74(2):365-73.
- Smith DB, Johnson KS. Single-step purification of polypeptides expressed in escherichia coli as fusions with glutathione S-transferase. Gene. 1988 Jul 15;67(1):31-40.
- Hopp TP, Prickett KS, Price VL, Libby RT, March CJ, Pat Cerretti D, et al. A short polypeptide marker sequence useful for recombinant protein identification and purification. Nat Biotech. 1988 print;6(10):1204-10.
- Hochuli E, Bannwarth W, Dobeli H, Gentz R, Stuber D. Genetic approach to facilitate purification of recombinant proteins with a novel metal chelate adsorbent. Nat Biotech. 1988 print;6(11):1321-5.
- Young CL, Britton ZT, Robinson AS. Recombinant protein expression and purification: A comprehensive review of affinity tags and microbial applications. Biotechnol J. 2012 May;7(5):620-34.
- Voss S, Skerra A. Mutagenesis of a flexible loop in streptavidin leads to higher affinity for the strep-tag II peptide and improved performance in recombinant protein purification. Protein Eng. 1997 Aug;10(8):975-82.
- Boozer C, Kim G, Cong S, Guan H, Londergan T. Looking towards label-free biomolecular interaction analysis in a high-throughput format: A review of new surface plasmon resonance technologies. Curr Opin Biotechnol. 2006 Aug;17(4):400-5.
- Bhikhabhai R, Sjoberg A, Hedkvist L, Galin M, Liljedahl P, Frigard T, et al. Production of milligram quantities of affinity tagged-proteins using automated multistep chromatographic purification. J Chromatogr A. 2005 Jul 1;1080(1):83-92.
- Bradford MM. A rapid and sensitive method for the quantitation of microgram quantities of protein utilizing the principle of protein-dye binding. Anal Biochem. 1976 May 7;72:248-54.
- Martin SR, Schilstra MJ. Circular dichroism and its application to the study of biomolecules. Methods Cell Biol. 2008;84:263-93.
- Zhang G, Annan RS, Carr SA, Neubert TA. Overview of peptide and protein analysis by mass spectrometry. Curr Protoc Protein Sci. 2010 Nov;Chapter 16:Unit16.1.
- Lorenzen K, van Duijn E. Native mass spectrometry as a tool in structural biology. Curr Protoc Protein Sci. 2010 Nov;Chapter 17:Unit17.12.
- Damian L. Isothermal titration calorimetry for studying protein-ligand interactions. Methods Mol Biol. 2013;1008:103-18.
- Some D. Light-scattering-based analysis of biomolecular interactions. Biophys Rev. 2013 Jun;5(2):147-58.
- Newman J, Egan D, Walter TS, Meged R, Berry I, Ben Jelloul M, et al. Towards rationalization of crystallization screening for small- to medium-sized academic laboratories: The PACT/JCSG+ strategy. Acta Crystallogr D Biol Crystallogr. 2005 Oct;61(Pt 10):1426-31.
- Backmark AE, Olivier N, Snijder A, Gordon E, Dekker N, Ferguson AD. Fluorescent probe for high-throughput screening of membrane protein expression. Protein Sci. 2013 Aug;22(8):1124-32.
- Hong P, Koza S, Bouvier ES. Size-exclusion chromatography for the analysis of protein biotherapeutics and their aggregates. J Liq Chromatogr Relat Technol. 2012 Nov;35(20):2923-50.
- Desjardins P, Hansen JB, Allen M. Microvolume spectrophotometric and fluorometric determination of protein concentration. Curr Protoc Protein Sci. 2009 Feb;Chapter 3:Unit 3.10.
- Ericsson UB, Hallberg BM, Detitta GT, Dekker N, Nordlund P. Thermofluor-based high-throughput stability optimization of proteins for structural studies. Anal Biochem. 2006 Oct 15;357(2):289-98.
Career Biographies
Rick Davies is an Associate Director within the Reagents and Assay Development department in the UK and leads a team of scientists responsible for generating recombinant proteins to support small molecule drug discovery projects across AstraZeneca. He started his industrial career in the late 1990s, extracting proteins from animal tissue to generate sequence information. He subsequently went on to work with recombinant proteins for almost all applications across all disease areas. This has involved embracing and responding to the many changes which have occurred in the field over the last 20 to 30 years and working on a wide range of target proteins.
Ian Hardern has a BSc (Hons) in Biological and Biochemical Sciences from The University of Salford and is currently a senior research scientist in the Discovery Sciences department at AstraZeneca. Starting his pharmaceutical R&D career in 1992, Ian has worked extensively within the field of protein expression, purification and analysis in support of small and large molecule research directed against oncology, respiratory, cardiovascular, infection and metabolism disease area targets. Ian currently holds the position of protein purification discipline lead in the Discovery Sciences department having previously held a similar position in the protein science group within the Oncology department.
Ross Overman joined AstraZeneca in 2002, entering the emerging field of Protein Engineering for Structural Biology after receiving his BSc (Hons) in Biochemistry and MRes in Biomolecular Sciences from the University of York. Initially undertaking a role as a molecular biologist he has since diversified into protein expression and purification and is currently an Associate Principal Scientist within the Discovery Sciences Reagents and Assay Development Group. Throughout his career he has continued to broaden his expertise by undertaking a part-time PhD with the University of Manchester. This project focussed on studying the biophysical, biochemical and structural differences between the EphB tyrosine kinase family and engineering their improved stability. Ross continues to coordinate multiple aspects of reagent provision for early-stage lead generation projects across a diverse set of target classes and has worked in many therapeutic areas.