Primary packaging materials for pharmaceutical freeze-drying: Moulded vs. serum tubing vials
Posted: 19 August 2010 |
Pharmaceutical freeze-drying is used to stabilise delicate drugs which are typically unstable in solution over a longer shelf life. The liquid formulation is converted into a solid, highly porous cake which can be easily reconstituted prior to administration. The majority of freeze-dried products in the pharmaceutical industry are used for parenteral application. This route of administration demands high quality for both the drug product and the primary packaging material. Today, glass vials are routinely used for freeze-dried products as they provide some indispensable characteristics. Depending on glass composition, surface treatment, processing and geometry, a vast number of different glass vials are commercially available for customers. Selection of the optimum vial for a given product seems to become more and more difficult as manufacturers of moulded and tubing glass have refined their products over the last decades to fulfil market needs.
Many biopharmaceuticals such as vaccines, hormones or monoclonal antibodies must be administered in injectable dosage form to be efficient for the medical attendance of a patient. Moreover, it has been shown that these drug molecules are frequently not stable in solution over a longer time period1. They are subjected to a variety of degradation reactions (e.g. hydrolysis, aggregation, fragmentation etc.) which can reduce biological activity of the drug and may increase potential negative side-effects for the patient2. Freeze-drying is a drying technology which allows the removal of (typically) water by means of sublimation, i.e. water is converted into ice and then removed as vapour without passing through the liquid phase3. Using this technology therefore allows a conversion of solutions containing labile and heat-sensitive drugs into a durable solid which can be quickly reconstituted even after a longer storage period3.
The freeze-drying process can be divided into three steps. In the first step (freezing), water is converted into ice and all solutes into solids3. The freezing step greatly influences the product morphology4. In the second step (denoted as ‘primary drying’), the chamber pressure is subsequently lowered and shelf temperature is slightly elevated to allow ice sublimation. Note that, for pharmaceutical freeze-drying, the relevant pressure range is between 0.07 to 0.27 mbar or 50 to 200 mTorr5. Here, the driving force for sublimation is the pressure difference between the vapour pressure of ice at the temperature of the frozen product (P0) and the chamber pressure (Pc)3. Higher product temperature means higher vapour pressure of ice and therefore higher sublimation rate so that the primary drying time can be greatly reduced6. A limiting factor for heat flow to the product is the critical formulation temperature. For crystalline materials, the critical formulation temperature refers to the eutectic temperature (Teut), for amorphous substances, this temperature is given in terms of the glass transition temperature of the maximally freezeconcentrated solute (Tg) or the collapse temperature (Tc)7. To maintain important product quality attributes such as an elegant cake appearance, fast and complete reconstitution and long storage stability, the product temperature profile during the drying process must stay below this critical temperature boundary7. In the final process step (secondary drying), the fraction of ‘unfrozen’ water (i.e. water of crystallisation or water embedded in the glassy matrix) is removed by diffusion and desorption effects. This way it is possible to obtain the desired residual moisture contents of the final product, typically less than one per cent5.
Glass for pharmaceutical packaging Glass is a multifunctional material which is omnipresent in our daily life, e.g. as construction material, in information and communication technology or as a container for foods and pharmaceuticals. Considering its chemical composition, glass is an inorganic product of fusion that forms a partially ordered network during cooling without crystallising8. A basic component of glass is silicon dioxide (silica, SiO2) where every silicon atom is surrounded by four oxygen atoms comparable to a tetrahedron. Since the fusion temperature of pure silica is extremely high, oxides are commonly added to obtain the desired physical and chemical properties8. For example, boron oxide (B2O3) improves the chemical resistance and aluminium oxide (Al2O3) makes glass less brittle. Sodium oxide (Na2O), calcium oxide (CaO) and potassium (K2O) oxide are added to lower fusion temperature and to increase formability9. Other oxides can be used to colour the glass, especially when the drug product is light sensitive, i.e. degrades when being exposed to short wavelength light8. Here, amber glass is routinely used for that purpose in the pharmaceutical industry8.
Opposed to the secondary packing material, the primary packaging material is in direct contact with the drug. This, in turn, leads to much higher demands and standards to protect the pharmaceutical product from environmental factors such as moisture, oxygen, microbial infestation, or the primary packing material itself. Physical and chemical interactions with the pharmaceutical product have to be taken into account and analytical testing is a requirement in almost all pharmacopoeias worldwide. Typical benefits for glass used as primary packing for pharmaceuticals have been reported in literature (e.g. glass containers are thermally stable for the purpose of sterilisation, impermeable and transparent)8,9.
Interactions with water, acid or alkali may lead to ion exchange in the glass network, a pH shift or even hydrolysis of the glass scaffold. Regarding chemical resistance, the European Pharmacopoeia distinguishes between three different types of glasses:10
- Type I: borosilicate glass with enhanced chemical resistance for sensitive drugs and multiple usage (common standard for parenterals). The superior chemical resistance of type I glass is a result of the lower Na2O content, a relatively high Al2O3 content and the additive B2O3
- Type II: belongs to the soda-lime glasses which show lower chemical resistance due to their composition. Because of a special treatment of the inner surface using for example SO2, type II glass gains chemical properties equivalent to type I glass but is only intended for single use. Due to this treatment, sodium is bound from the glass and the glass surface is ‘dealkalised’. It may contain acid or neutral aqueous solutions for parenteral application
- Type III: is a soda-lime glass with low hydrolytic resistance used for non-aqueous parenteral dosage forms, powders for production of parenteral dosage forms (except lyophilised drugs) and products for non-parenteral application
For convenience, the composition of the different glass types is given in Table 1.
Weight (%) | Soda-lime glassblown | Borosilicate glassblown | Borosilicate glasstubing |
SiO2 | 73.0 | 67.8 | 80.4 |
B2O3 | – | 13.6 | 12.9 |
Al2O3 | 1.8 | 5.8 | 2.6 |
CaO | 10.7 | 1.1 | < 0.05 |
MgO | 0.4 | <0.1 | < 0.05 |
BaO | – | 2.4 | – |
Na2O | 13.5 | 8.3 | 4.0 |
K2O | 0.3 | 0.8 | – |
Manufacturing of vials
At the beginning of the manufacturing of vials, the raw materials are weighed and mixed according to the desired glass composition8. The mixture is fed to the furnace in discrete batches and is molten at temperatures around 1500°C. The glass melt is withdrawn continuously and distributed to the forming equipment by the feeder. Regarding the forming and processing, one can differentiate between ‘blown’ containers (also called moulded vials) and vials made from tubes8.
To form moulded vials, gobs of molten glass are delivered to the blank moulds of the IS machine. Here, the finish and the blank shape are formed either by compressed air (blow & blow process) or by an inserted plunger (press & blow process). Afterwards, the blank is transferred to the finish mould where compressed air is applied to blow the container to its final form. At this point, the temperature of the containers is still around 500°C. They are transported on a conveyor belt to the annealing lehr where they are reheated and then cooled uniformly in order to release stresses arising during the manufacturing process. At the cold end of the annealing lehr, the containers are subjected to several optical inspections to guarantee the quality of the bottles, vials, etc.
The production of tubing vials is divided into two main operations. First, the glass tubes have to be drawn out of the molten glass. One example is the ‘Danner process’ where the glass melt flows onto a slowly rotating ceramic hollow cylinder (Danner pipe) which is placed in an inclined position. Compressed air flows through the cylinder to form the tube. By varying the blowing pressure and the rate of drawing, a wide range of diameters and wall thicknesses can be processed with extremely high precision and uniformity. In a second step, the glass tubes are converted into the final form (e.g. ampoules, vials, syringe bodies, cartridges etc.) by means of heat and special tools. Most tubing-made containers are made of borosilicate glass, but in general, borosilicate and soda-lime glass can be processed with both manufacturing techniques. Independent from the production process, all glass containers can be treated at the inner or outer surface to improve the chemical and physical properties.
Vials for freeze-drying: impact on process and product performance
As mentioned above, the heat flow (dQ/dt) to the product is a critical parameter during lyophilisation as it governs the effective product temperature during drying. The properties of the container greatly affect the heat transfer from the shelf (the heat ‘source’) to the product. Here, the container imposes the greatest resistance to heat flow and the uncertainty of homogenous heat transfer for the entire batch. Moreover, the quality and / or the design of a glass container can be critical for vial breakage. A proper choice of the vial itself can therefore be decisive for the success and the profitability of a freeze-drying cycle. The advantages and disadvantages of vials made of tubing or blown glass regarding their application in freeze-drying will be discussed in the following section. Table 2 compares the most important parameters.
Table 2. Comparison between moulded containers and tubing containers (+: positive, -: negative)
Tubing | Molded | |
visual appearance | ++ | + |
thickness of wall and bottom | ++ | + |
weight | + | – |
heat transfer | ++ | + |
resistance to vial breakage | – | + |
nominal volume less than 5 mL | + | – |
syringes, cartridges, ampoules | + | – |
surface treatment inside/outside | + | + |
price | – | + |
A freeze-drying cycle can be considered as ‘optimum’ if the effective cycle time is short and the resulting product of high quality5. A typical approach to cut process time is to reduce the primary drying phase by maintaining product temperature as close as possible to the collapse temperature11 or even within the ‘microcollapse regime’12. In order to take a real advantage of the maximum possible heat flow from the shelf at a given chamber pressure, not only the knowledge of the collapse temperature but also information about the heat transfer characteristics of the vial is mandatory. Note that the actual product temperature profile is mainly influenced by shelf temperature, chamber pressure and heat transfer into the product6. The routine procedure to modulate product temperature is to adjust the shelf (inlet) temperature, rather than changing the chamber pressure5. The shelf temperature adjustment allows a better control of the heat flow from the shelf to the product, a change in chamber pressure might negatively influence the batch drying homogeneity. Shelf temperature changes are specifically preferable in cases where the vial allows a higher heat transfer rate from the shelf to the product5. In other words, if a given container system imposes very little heat flow resistance from the heat ‘source’ to the heat ‘sink’, even small step changes in shelf temperature result in small changes in product temperature. This is highly desirable since this situation offers 1) an additional ‘margin’ for a further increase in shelf (inlet) temperature and 2) allows better fine tuning of product temperature at a given stage of drying. As an additional point for consideration, a low resistance to heat flow can also reduce drying heterogeneity among vials.
Apart from heat transfer characteristics, vial breakage is the second critical factor impacted directly by the choice of the container system. From an economic point of view, vial breakage during production of an expensive drug (e.g. an antibody) is extremely undesirable since it further decreases the effective batch-size, extends the clean-in-place (CIP) procedure and wastes drug resources. If cytotoxic products are processed, hazard potential is increased dramatically and extensive cleaning procedures have to be conducted. Breakage is the result of an unbalanced relation of stress and strength in the glass vial13. Stress can be induced mechanically e.g. during loading of the freeze-dryer or during automatic stoppering. Thermal strain emerges when the glass container is subjected to fast heating or cooling or when heat distribution in the container body is heterogeneous. In addition, a certain stress may also rise from the formulation itself. As a well known example, re-crystallisation of amorphous during early primary drying can result in a tear-off of the vial bottom14. In addition, vial breakage has been observed for high-concentrated protein drugs at high fill volumes where the cake ‘shrinks’ during primary drying and the volume contraction imposes a mechanical stress to the vial wall. To reduce risk of vial breakage, these stresses should be kept at a low level from process and formulation side but also by choosing an appropriate container system. Here, slightly elevated thickness of wall and bottom is beneficial.
Evaluation of the heat transfer characteristics of vials
Three heat transfer mechanisms have been reported in the literature:15
- direct conduction from shelf to vial at points of direct contact (dependent on vial design)
- conduction through the gas between shelf and vial bottom (dependent on pressure and vial design)
- radiative heat transfer (dependent of freezedryer type and design)
A well-established procedure to compare heat transfer characteristics of different vial types is the determination of the vial heat transfer coefficient Kv (cal·s-1·cm-2·°C-1) by performing sublimation tests with pure water at various chamber pressure settings. Kv is defined as the ratio of the area normalised heat flow to the temperature difference between heat source and heat sink and can be described with the following equation (Equation 1):16
Eq.1
where ΔHs is the heat of sublimation of ice (660 cal/g, temperature dependent), Av the vial outer cross-sectional area (cm2), Ts the shelf surface temperature and Tb the product temperature at the bottom-centre of the vial (°C). Mass loss (dm/dt) is determined gravimetrically by weighing (all) filled vials prior to and after the sublimation test16. As the calculation of Kv refers to a steady state model17 the ramping phase at the very beginning of the primary drying should be excluded from the determination of mass loss. However, many studies published in the literature did not necessarily follow this rule. Besides using the traditional gravimetric procedure, the mass flow rate (g/s) has also been determined by Tunable Diode Laser Absorption Spectroscopy (TDLAS)18,19. Due to this noninvasive method, water vapour concentration and vapour flow velocity in the spool piece between the freeze-dryer chamber and condenser can be measured in real-time18. The Kv values evaluated at different pressure settings are then analysed by non-linear regression analysis using Equation 2:15,16
Eq.2
Here, P is the chamber pressure (Torr), KC is the sum of the heat transfer through direct conduction and radiation, KD is the pressure dependent term and is directly proportional to the mean separation distance (lv) between the vial bottom and the shelf surface. KP is a constant for vials (3.32·10-3 cal/s cm2°C Torr)15. The fitted curve then provides KC and KD values which show the influence of the geometry of the vial bottom on heat transfer.
Desired features of an optimised vial for freeze-drying include a thin bottom, a high contact area and low bottom concavity. Note that a certain concavity is needed for the required mechanical stability13. Historically, these attributes are related to tubing vials which have been found superior to moulded vials regarding Kv values16. In 2008, Kuu and co-workers compared six different vials on the basis of Kv values determined using TDLAS20. In a pressure range from 25 to 400 mTorr, the moulded vial type had the lowest Kv values. Tang5 and Pikal16 stated that tubing vials have higher heat input through direct contact and more heat input through gas conduction relative to moulded vials. Pikal also stated that the thickness of the vial bottom is less important16. A possible explanation for the comparatively bad performance of the moulded vials reported in the cited literature5,16 is the greater diameter. Note that, from a manufacturing perspective, the bottom concavity and thickness are elevated for bigger vials to ensure their mechanical rigidity.
Moulded vs. tubing vials for freeze-drying: recent developments
Two of the biggest manufacturers of vials, SCHOTT forma vitrum AG and SGD S.A., recently introduced new product lines specifically made for freeze-drying applications. According to SCHOTT forma vitrum, their new serum tubing vials (denoted as TopLyoTM) feature an optimised geometry for enhanced heat transfer and a reduced risk of vial breakage21. In addition, during the SCHOTT PICVD (Plasma Impulse Chemical Vapour Deposition) process, thin layers of hydrophobic coating are applied to the inner surface. Conventionally, the inside of vial is lined with silicon oil (siliconisation)23. This procedure bears the risk of creating sub-visible (silicon) particles in solution or even elevated aggregation of a protein. The PICVD procedure is expected to avoid formation of a cake ‘crown’, cake disruptions, risk of vial breakage and can increase the extractable volume of the reconstituted solution from the vial22. The PICVD process promises a covalent bond between glass and coating to guarantee inertness of the inner surface22.
In contrast, SGD S.A. has newly developed a moulded vial (EasyLyoTM) for small volumes (e.g. 5 and 10mL) suitable for freeze-drying. Note that moulded vials were traditionally used for higher fill volumes (> 50mL) in the pharmaceutical industry. The optimised vial geometry improves heat transfer and the cosmetic look of the dried cake24. Furthermore, the EasyLyoTM vial is 30 per cent lighter compared to a standard moulded vial with reduced risk of vial breakage compared to a standard serum tubing vial25.
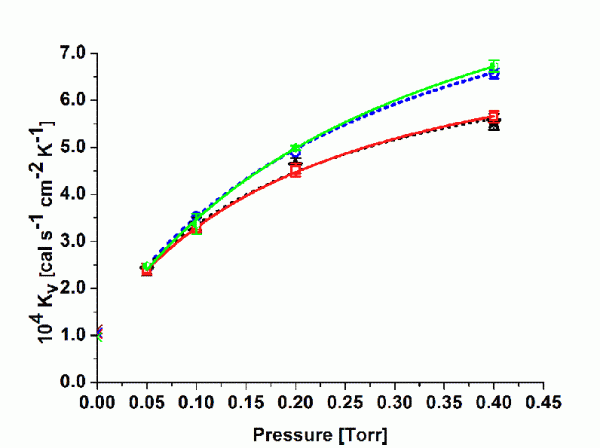
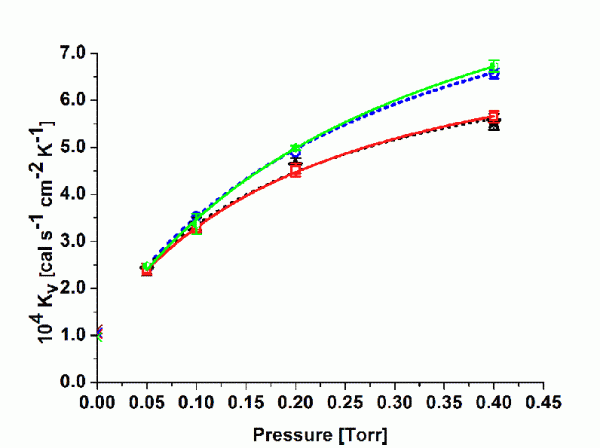
Figure 1 Kv values at different pressure settings (centre vials, average from two experiments per pressure setpoint). Symbols represent: upper solid line = curve fit TopLyoTM vial, upper dotted line = curve fit ‘standard serum tubing’ vial, lower solid line = curve fit EasyLyoTM vial, lower dotted line = curve fit ‘standard moulded’ vial.
Vials for freeze-drying: heat transfer characterisation
Independent studies from our group recently investigated the heat transfer characteristics of some of the newly introduced commercial products by performing sublimations tests with pure water at various pressure settings (‘gravimetric’ procedure). Surprisingly, some of the ‘conventional wisdom’ mentioned above could only be confirmed in parts.
Table 3. Fitting parameters for the four vial types
EasyLyoTM average 1/2 | Molded average 1/2 | Serum tubing average 1/2 | TopLyoTM average 1/2 | |
KC | 1.03∙10-4 | 1.13∙10-4 | 1.06∙10-4 | 0.970∙10-4 |
KD | 4.67 | 4.91 | 3.50 | 3.27 |
KP | 33.2∙10-4 | 33.2∙10-4 | 33.2∙10-4 | 33.2∙10-4 |
Kv values were determined for the 10mL SCHOTT forma vitrum TopLyoTM vial and for the 10mL SGD EasyLyoTM vial as well as for a 10mL standard tubing vial (Thüringer Pharmaglas) and a 10mL standard moulded vial (SGD S.A.). As mentioned above, the calculation of Kv should refer to steady state conditions during primary drying. Additional tests were performed to determine the mass of water removed during the ramping interval for every pressure setting until steady state conditions were achieved. It was therefore possible to solely determine the amount of water sublimed during the steady state26. Figure 1 illustrates non-linear regression analysis (Microcal Origin) according to Equation 2 for the four vial types (centre vials only, average of two series of experiments) in the investigated pressure range (50 to 400 mTorr). As expected, Kv values increased with elevated pressure settings due to enhanced heat transfer through gas conduction. However, the difference between tubing and moulded vials was lower than expected, in particular in the relevant pressure range in pharmaceutical freeze-drying (50 to 200 mTorr). Both of the new vials showed no clear advantage in heat transfer relative to the standard vials. The fitting parameters are given for convenience in Table 3. KC represents the heat input from conduction via points of direct contact, whereas KD stands for the pressure dependant part, i.e. conduction through the vapour between shelf and vial bottom. Bottom concavity was found to be lower for the tubing type (lower KD values) but there was no indication of an elevated contact area (KC values are in the same range for all vial types). It is worthwhile to mention that SCHOTT forma vitrum TopLyoTM revealed a very consistent and homogenous heat transfer of all vials throughout the batch. It is well-known from literature that ‘edge’ vials reach higher Kv values compared to centre vials due to additional heat flow by radiation from chamber door and wall27. The heat transfer characteristics of the SCHOTT forma vitrum TopLyoTM vials were, however, found to be extremely balanced between these to vial positions, in particular at higher pressure settings (see Figure 2).
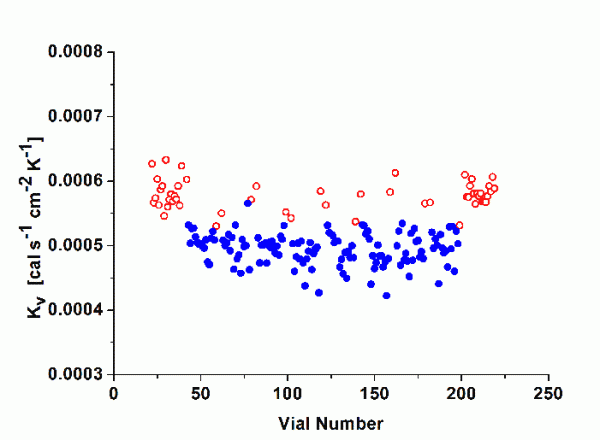
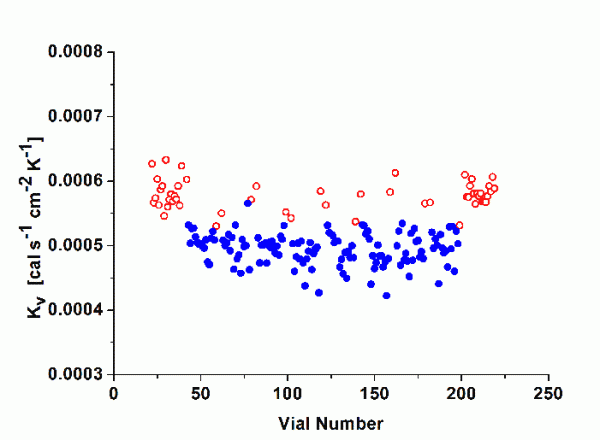
Figure 2 Kv values according to vial number at a chamber pressure of 200 mTorr and a shelf temperature of -10°C, SCHOTT forma vitrum TopLyoTM. Symbols represent: empty circles = edge vials, filled circles = centre vials.
Conclusions
For many pharmaceuticals that are unstable in aqueous solution, freeze-drying is the method of choice to assure quality over a longer shelf life. Besides optimisation of the formulation and the freeze-drying cycle, the container can also greatly impact the success of the process. Historically, freeze-drying of small-volume parenterals was a domain of serum tubing vials. Such vials can be processed in small sizes with high precision and thin bottom and walls. To reduce the risk of vial breakage, vial geometry was improved. In contrast, blown glass con tainers were mainly considered for larger volumes (50mL and more) as they were more stable. It appears that manufacturing processes and machines for moulded vials were gradually improved such that it is possible today to produce moulded vials with even small volumes and uniform dimensions. The ‘perfect’ vial for freeze-drying should, from a process perspective, combine a maximum heat transfer and minimum vial breakage. While this was a question of the manufacturing process (i.e. tubing or blown glass) over the last years, it seems to become more and more a matter of glass composition (e.g. clear or amber) and a fine-tuning of vial geometry.
References
1. M. J. Pikal. Mechanism of Protein Stabilization During Freeze-Drying and Storage:The Relative Importance of Thermodynamic Stabilization and Glassy State Relaxation Dynamics. In: Freeze-Drying/Lyophilization of Pharmaceutical and Biological Products (L. Rey and J. C. May, Eds), Marcel Dekker, New York (2004).
2. F. Franks. Freeze-drying of bioproducts: putting principles into practice. Eur J Pharm Biopharm 45:221-9 (1998).
3. S. L. Nail and L. A. Gatlin. Freeze Drying: Principles and Practice. In: Pharmaceutical Dosage Forms: Parenteral Medications, Vol. 2 (K. E. Avis, H. A. Liebermann and L. Lachman, Eds) Marcel Dekker, New York (1993).
4. J. J. Schwegman, L. M. Hardwick, M. J. Akers.Practical Formulation and Process Development of Freeze-Dried Products. Pharm Dev Technol 10:151-173 (2005)
5. X. Tang and M. J. Pikal. Design of freeze-drying processes for pharmaceuticals: practical advice. Pharm Res 21:191-200 (2004).
6. M. J. Pikal. Freeze Drying. Encyclopedia of Pharmaceutical Technology.Marcel Dekker, New York (2002).
7. Carpenter, J.F., Pikal, M.J., Chang, B.S., Randolph, T.W. Rational Design of Stable Lyophilized Protein Formulations: Some Practical Advice. Pharm. Res.14:969-75 (1997).
8. R. P. Abendroth and R. N. Clark. Glass Containers for Parenterals. In: Pharmaceutical Dosage Forms: Parenteral Medications, Vol. 1 (K. E. Avis, H. A. Liebermann and L. Lachman, Eds), Marcel Dekker, New York (1992).
9. K. H. Bauer, K-H. Frömming, C. Führer. Primärpackmittel. In: Lehrbuch der Pharmazeutischen Technologie, Wissenschaftliche Verlagsgesellschaft mbH, Stuttgart (2006)
10. Europäische Arzneibuchkommission. Europäisches Arzneibuch, 6. Ausgabe, Govi Verlag, Eschborn (2008).
11. M. J. Pikal. Freeze-drying of proteins part II: formulation selection. BioPharm 3:26-30 (1990)
12. R. E. Johnson, M. E. Oldroyd, S. S. Ahmed, H. G. Gieseler, L. M. Lewis. Use of manometric temperature measurements (MTM) to characterize freeze-drying behavior of amorphous protein formulations. J. Pharm. Sci. 99:2863-2873 (2010).
13. J. Thürk and P. Knaus. Advanced Glassware for Freeze-Drying. In: Freeze-Drying/ Lyophilization of Pharmaceutical and Biological Products ( L. Rey and J. C. May, Eds), Marcel Dekker, New York (2004).
14. N. A. Williams, Y. Lee, G.P. Polli, T. A. Jennings. The effects of cooling rate on solid phase transitions and associated vial breakage occurring in frozen mannitol solutions. J. Parenter. Sci. Technol. 40:135-141 (1986).
15. M. J. Pikal. Use of laboratory data in freeze drying process design: heat and mass transfer coefficients and the computer simulation of freeze drying. J Parenter Sci Technol 39: 115-39 (1985).
16. M. J. Pikal, M. L. Roy, and S. Shah. Mass and heat transfer in vial freeze-drying of pharmaceuticals: role of the vial. J Pharm Sci 73: 1224-37 (1984).
17. S. Rambhatla, M. J. Pikal. Heat and Mass Transfer Issues in Freeze-drying Process Development. In: Lyophilization of Biopharmaceuticals (H. R. Constantino, M.J. Pikal, Eds), AAPS Press (2004).
18. H. Gieseler, W. J. Kessler, M Finson, S. J. Davis, P. A. Mulhall, V. Bons, D. J. Debo, M. J. Pikal. Evaluation of tunable diode laser absorption spectroscopy for inprocess water vapor mass flux measurements during freeze drying. J Pharm Sci 96(7):1776-1793 (2007).
19. S. C. Schneid, H. Gieseler, W. J. Kessler and M. J. Pikal. Non-invasive product temperature determination during primary drying using tunable diode laser absorption spectroscopy. J Pharm Sci (2008).
20. W. Y. Kuu, S. L. Nail, and G. Sacha. Rapid determination of vial heat transfer parameters using tunable diode laser absorption spectroscopy (TDLAS) in response to step-changes in pressure set-point during freeze-drying. J Pharm Sci 98: 1136-54 (2009).
21. Press Release 1.12.2009, SCHOTT homepage: http://www.schott.com/english/news/press.h tml?NID=2690 (accessed June 2010).
22. SCHOTT homepage: http://www.schott.com/ pharmaceutical_packaging/english/ download/ special_solutions.pdf (accessed June 2010).
23. R. J. Harwood, J. B. Portnoff. E. W. Sunbery. The processing of Small Volume Parenterals and Related Sterile Products. In: Pharmaceutical Dosage Forms: Parenteral Medications, Vol. 2 (K. E. Avis, H. A. Liebermann and L. Lachman, Eds), Marcel Dekker, New York (1993).
24. SGD homepage. http://www.sgdpharma. com/spip.php?rubrique73&lang=en (accessed July 2010).
25. C. Wagner. Effect of Solute, Concentration and Solution Fill Height on Incidence of Vial Breakage During Freezing and Freeze Drying: A vial parameter study. PDA, Frankfurt (2009).
26. S. Rutzinger, H.Gieseler. Evaluation of the Heat Transfer Characteristics of a New Container System for Pharmaceutical Freeze-Drying. 7th World Meeting on Pharmaceutics, Biopharmaceutics and Pharmaceutical Technology, Valetta, Malta (2010).
27. S. Rambhatla and M. J. Pikal. Heat and mass transfer scale-up issues during freeze-drying, I: atypical radiation and the edge vial effect. AAPS PharmSciTech 4: E14 (2003).
About the Authors
Susanne Hibler
Susanne Hibler has been a licensed pharmacist in Germany since 2008 and started a PhD in the Freeze Drying Focus Group in the same year. Her major research interest is freeze dry microscopy of biopharmaceuticals, in particular the transferability of collapse temperature data to freeze-drying cycles and FDM as an instrument to support Quality by Design (QbD) and process analytical technologies (PAT) in freeze-drying. In addition, she investigates the heat transfer characteristics of different vial systems for pharmaceutical freeze-drying.
Contact the Author
email: [email protected]
Dr. Henning Gieseler
Dr. Gieseler has been a licensed pharmacist in Germany since 2000 and received his PhD in 2004 at the Department of Pharmaceutics, University of Erlangen (Professor G. W. Lee). After a post-doctoral research period at the School of Pharmacy, University of Connecticut (Professor Michael J. Pikal) until early 2006, he returned to his former Department in Erlangen. He is currently the head of the Freeze Drying Focus Group in the Department of Pharmaceutics. Dr. Gieseler’s research interests deal with various aspects of freeze-drying, in particular formulation design for biopharmaceuticals and nanomaterials, process design and optimisation of freeze-drying cycles (including PAT) and scale-up. He has authored and co-authored numerous publications in the field of freeze-drying over the last years and is a well-known expert in this specific field of interest.
Contact the Author
email: [email protected]