Microfluidic techniques for cancer therapies
Posted: 29 June 2017 | Jeffrey T Borenstein | Draper | 1 comment
Perhaps the most significant challenge in all of medicine is the need for more effective treatments for cancer, which causes one of every four deaths in the United States and bears a direct cost to our healthcare system of close to $100bn annually.
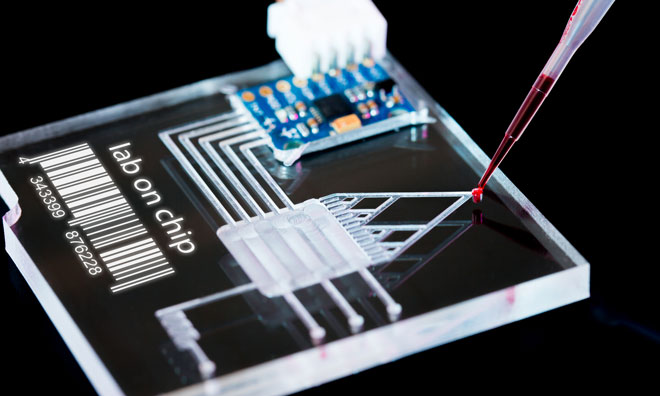
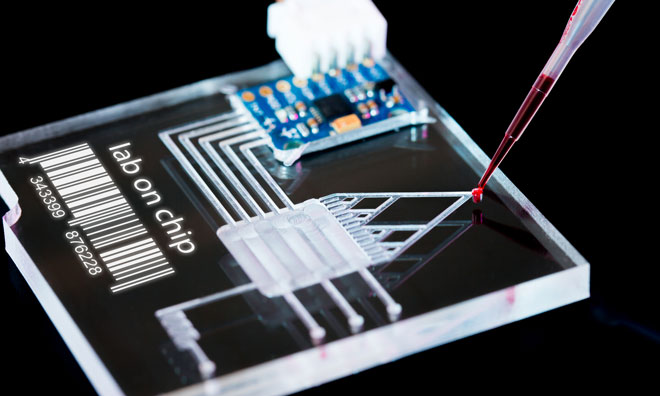
Over the past several decades, therapeutic approaches to cancer have developed around surgery, radiation and chemotherapy, yet with over 600,000 new cases of cancer per year in the United States, one-year survival rates for some cancers are below 20%.
In response to this tremendous challenge, new approaches to cancer treatment have been emerging, ranging from hormone therapies to treat breast and prostate cancer, to targeted therapies aimed at inhibiting angiogenesis or cell growth, to a host of novel immunotherapies that harness the body’s immune cells to fight the cancer. Since up to 90% of cancer deaths are due to metastasis of the primary cancer site,1 early detection and strategies to prevent metastasis have also risen to the fore. In this article, we focus on cancer therapy and the role that a new class of microfluidic technologies is playing in contributing to tools and platform technologies that refine and accelerate cancer treatments in ways that extend well beyond conventional laboratory systems.
Microfluidics technologies have been in development for the past three decades, with early progress and demonstrations focusing on lab-on-a-chip systems for bioanalysis and clinical diagnostics.2 Over the past decade, the field has branched in several new and exciting directions, many of which have the potential to address longstanding challenges in health care, such as the need for new tools to assess the safety and efficacy of candidate therapeutics in preclinical development.3
Here, we will focus on three applications of microfluidics technologies that have the potential to revolutionise cancer therapeutics, each of which is currently being developed by research scientists at Draper and collaborating institutions. These three approaches include organ-on-chip models for diseases and for mimicking processes such as tumour intravasation in scalable microfluidic formats, microfluidic systems capable of ex vivo recapitulation of tumour-immune and tumour-drug interactions using human patient biopsy samples, and safer and more efficient microfluidic systems for manipulating stem cells and immune cells for adoptive cell transfer (ACT) technologies.
Microfluidic organ-on-chip systems
The drug development pipeline is struggling due to many factors, among them the poor predictive power of preclinical models for assessing the safety and efficacy of emerging therapeutic compounds. Currently, preclinical studies rely on in vivo animal studies and conventional cell culture models, neither of which are particularly effective in predicting human responses to drug therapies. A limitation of in vivo models is that mechanistic studies are difficult in living organisms, due to the inability to precisely monitor and control the microenvironment. The latter method typically relies upon transformed cell lines cultured in two dimensions in multiwell plates – an approach that does not effectively recapitulate the physiologic microenvironment.
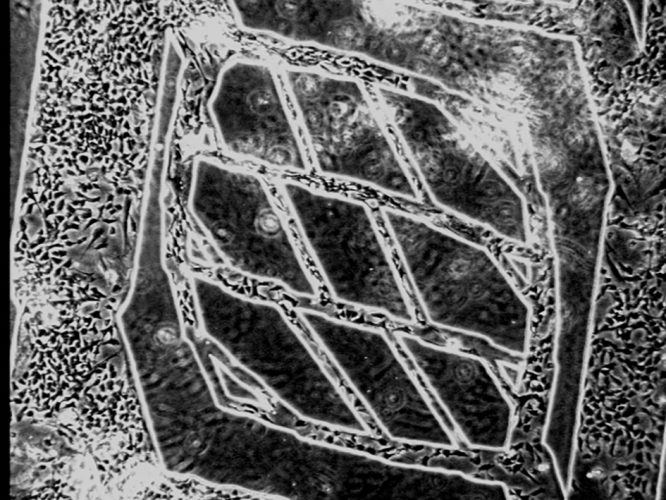
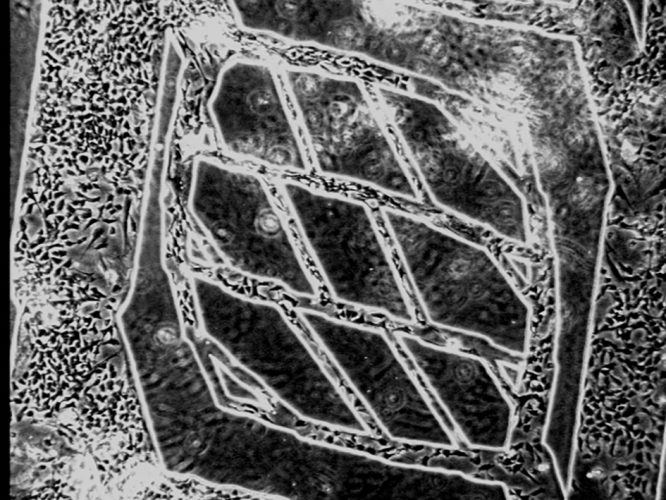
Figure 1: Microfluidic engineered vascular network comprising microfabricated branching network of channels seeded with endothelial cells
These limitations have motivated the development of microfluidic organs on chips, a technology in which human primary cells are seeded in microfabricated constructs capable of mimicking salient features of the tissue microenvironment. One of the most critical features of microfabrication technology relates to its ability to recapitulate the chemical and mechanical microenvironment within human organs at a relevant size scale of tens of microns, equivalent to the physiological features and structures such as blood capillaries, renal proximal tubules, liver sinusoids and other key targets for disease and safety models. A key component of these systems is the branching architecture of blood vessels, first simulated using microfluidic technologies 15 years ago4 when silicone polymers were micromoulded against high-precision silicon wafer masters, bonded and seeded and cultured with endothelial cells for periods of up to four weeks. An image of a branching blood vessel network seeding with endothelial cells is shown in Figure 1.
A key aspect of mimicking the tumour microenvironment is the ability to recapitulate the process of tumour intravasation, where tumour cells enter the bloodstream, in a microfluidic system.5 An image of such a system is shown in Figure 2, where an endothelialised side channel is juxtaposed next to a compartment containing tumour cells, with an intervening gel region designed to mimic the three-dimensional extracellular matrix. Advantages of this system include the ability to image processes in real time – and at high resolution – and precise monitoring of the endothelial barrier function, a critical aspect of simulating the tumour intravasation process. In addition to enabling greater understanding of the processes involved in intravasation, this system may support applications in drug screening and ultimately in precision medicine as patient-tailored therapies are developed.
Microfluidic tumour biopsy evaluation systems
The field of immunotherapy has been around for more than a century, but only recently has the field experienced rapid growth upon the realisation that a class of compounds known as immune checkpoint inhibitors can treat many cancers not amenable to standard chemotherapeutic approaches.6 The immune system contains many checkpoints that confer self-tolerance and protect against cell and tissue damage otherwise possible from the activity of immune cells such as lymphocytes. Tumours often appropriate these checkpoint pathways by establishing ligand-receptor links that serve to block the immune response.
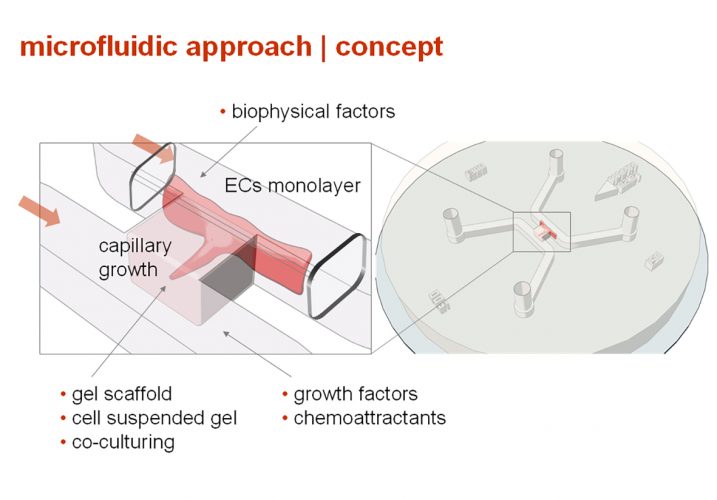
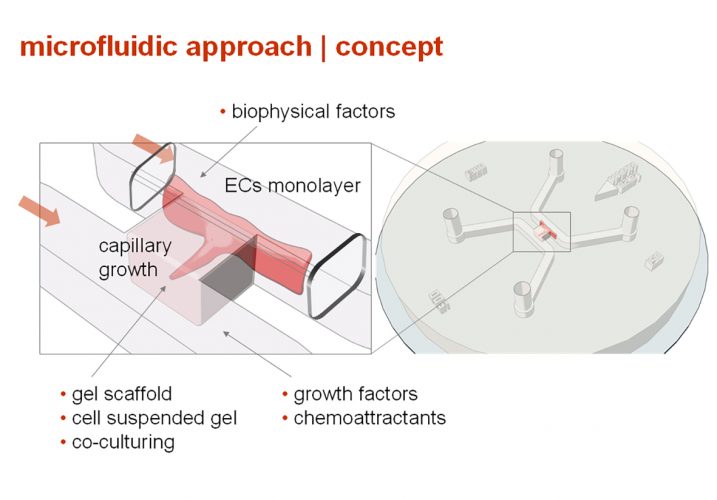
Figure 2: Model for tumour intravasation comprising adjacent regions of tumour cells, extracellular matrix and an endothelialised microvascular channel
Over the past decade, dramatic advances in immune checkpoint inhibitor therapies have been made, with a series of classes of antibodies known as CTLA4 and PD-1 emerging as potential treatments that block the protective pathway that many tumours have established to shield themselves from a robust immune response. A primary challenge in this field involves limitations in preclinical models used to predict the efficacy of candidate therapeutic proteins on human tumours. Typically, pharmaceutical companies rely upon syngeneic mouse models, genetically engineered mouse models, and humanised mouse models to assess response to immune checkpoints or combinations with small molecules. However, these mouse models are often poor predictors of clinical performance, can be very expensive, and do not permit mechanistic studies where real-time tumour progression can be monitored and assayed as a function of local environmental parameters.
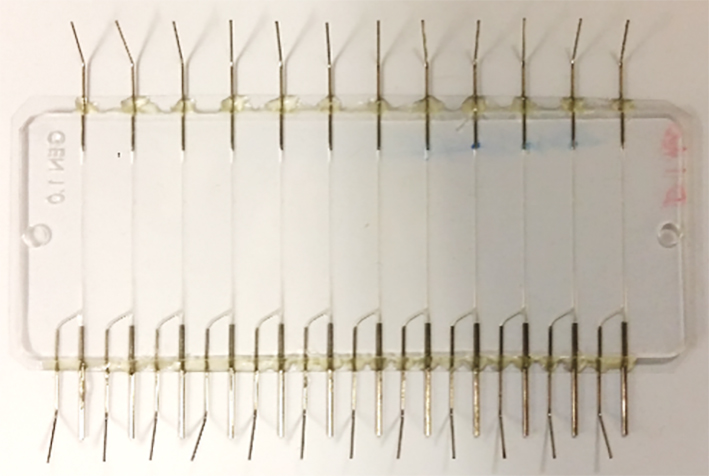
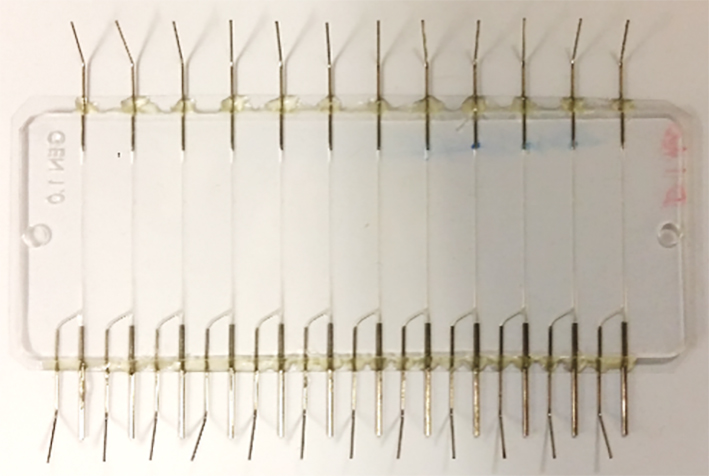
Figure 3: 12-channel PPACT microdevice simulating the interaction between tumour-infiltrating lymphocytes TILs) and tumour fragments with real-time imaging and quantification
In response to the above-mentioned limitations, human tumour histoculture has emerged as a potential way to provide preclinical assessment of the efficacy of PD-1 inhibitors, and many efforts and platforms are now being reported. However, these systems are generally static in nature, comprising multiwell plates containing tumour fragments or spheroids that are degrading over periods of hours to days while being tested against candidate compounds. Further, interactions between patient immune cells and tumour samples are difficult to recapitulate in static systems, due to poor control of the interaction cross-section between tumour-infiltrating lymphocytes (TILs) and tumours across replicates in statistically significant populations.
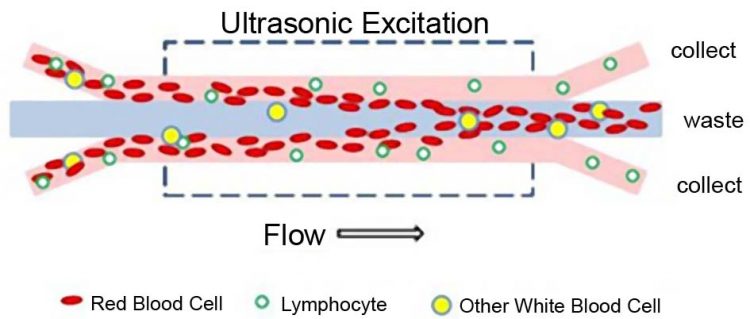
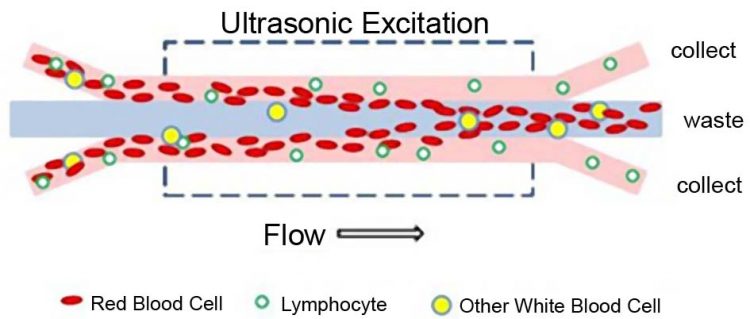
Figure 4: Acoustic separation system for enriching populations of lymphocytes from whole blood for CAR-T and other cell therapies
Recently, a new approach to gauging human response, known as personalised predictive assay for cancer therapeutics (PPACT), has emerged7,8 in which mouse or human tumour fragments are loaded into microchannels containing a trapping region that entrains the tumour while oxygenated media is perfused through and past the tumour. A 12-channel multiplex device has been established that is capable of exposing tumours to populations of TILs in a controlled manner for periods of up to a week or more (see Figure 3). To screen various candidate treatments, TILs may be presented to tumours as harvested from the tumour samples or following exposure to various combinations of immune checkpoint inhibitors and small molecules. The microfluidic PPACT system permits real-time quantitative imaging and assaying of TIL infiltration and tumour death in each channel, providing far greater insight and precision than has been previously available in existing preclinical tools.
Adoptive cell therapies using microfluidic processing technologies
One of the most significant recent advances in the treatment of blood cancers – and potentially more broadly in solid tumours – has been the emergence of adoptive cell therapies (ACT), principally CAR-T (chimeric antigen receptor-T cell technology) therapy.9 This process comprises: the extraction of a blood sample from a cancer patient, typically with acute lymphoblastic leukaemia (ALL) or lymphoma; separation of the T cell population from the whole blood sample; activation and genetic manipulation using nucleic acid delivery to programme the cells to attack the cancer; expansion of the genetically modified T cells as needed, and final formulation. These steps are currently carried out using labour-intensive and operator-dependent processes that may not be fully standardised and are extremely expensive and time-intensive. Such manufacturing challenges remain the most significant barrier to the expanded use of CAR-T therapy to treat a broadening range of cancers, despite very exciting and promising results in halting cancer progression and, in some cases, apparent full remission of late-stage intractable disease in many patients.
Current processing includes steps such as Ficoll-Paque separation of lymphocytes from blood samples, which has limitations in efficiency and is difficult to automate. For nucleic acid delivery, viral transduction of T cells is often used – a process that entails high use of extremely expensive virus delivery vehicles, and often results in poorly controlled levels of viral transfer to T cell populations. Transduction often invokes the use of centrifugation, a mechanically vigorous and potentially damaging step that processes cells in a bulk format and has significant limitations in transduction efficiency.
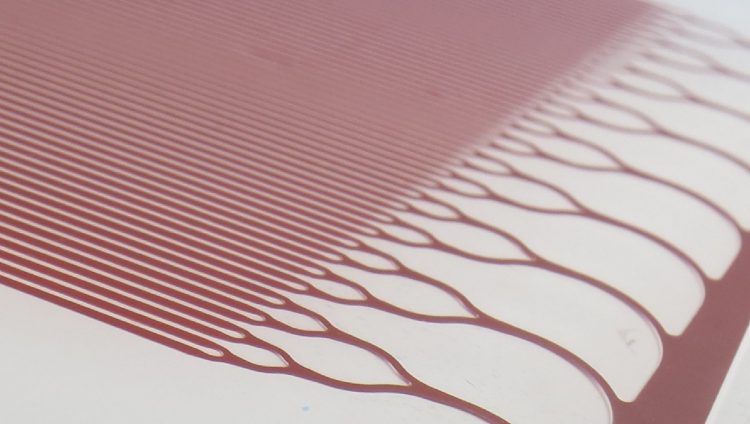
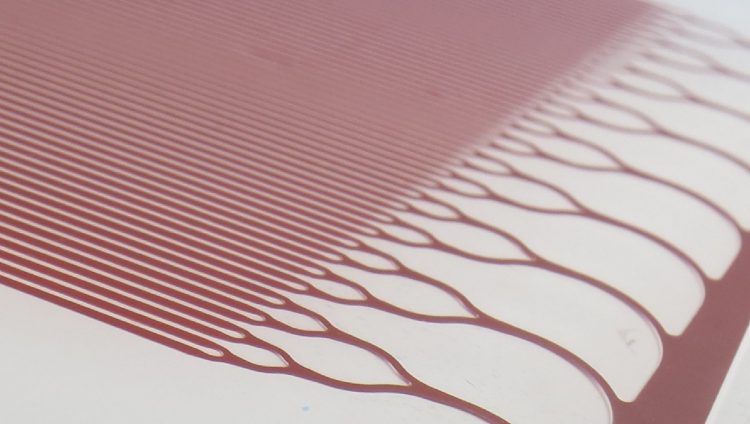
Figure 5: Trilayer microfluidic device for viral transduction of lymphocytes using transmembrane pressure to control interactions between cells and virus particles
Microfluidics technologies can be brought to bear on many, if not all, of the steps in the CAR-T production process listed above. Two in particular – cell separation and nucleic acid delivery – have been demonstrated using highly innovative processing techniques based on microfluidic devices. Examples of these techniques are shown for illustration in Figures 4 and 5 , respectively. Recent progress in acoustophoresis, in which acoustic waves are generated in microchannels to separate blood components by size and density, and, to a lesser degree, compressibility,10 has been shown to be highly effective in sorting and enriching populations of lymphocytes for later processing for CAR-T therapy. The process has the advantages of being continuous and therefore adaptable to accommodate a wide range of cell numbers and volumes and does not involve centrifugation.
For viral transduction, processes such as static transduction and spinoculation (centrifugation of cells and viral vector) are often inefficient, requiring large amounts of expensive viral vector, which can be challenging to manufacture. These problems are being addressed by a novel approach that uses a microfluidic trilayer device,11 in which cells and virus particles are introduced into a branching network of channels and are sequestered within microfabricated zones in which they are held by gentle transmembrane pressures. These can be precisely controlled and designed to target the specific exposure times and spatial arrangements of the cells and viruses. This microfluidic technology is amenable to scale-up to achieve the necessary cell numbers and throughput rates required for efficient and cost-effective clinical therapy.
Summary
Here we have described several emerging microfluidic tools and platforms aimed at advancing the process of developing cancer treatments. Common features of these systems are that they are designed to replace conventional macroscale technologies that lack the precision and control necessary to either mimic the microenvironment for simulating tumour-cell interactions, or to safely manipulate populations of cells in preparation for administration of cell therapies. A key aspect of the microfluidic systems we have described, beyond their precision and control capabilities, is their scalability toward levels of relevance for human therapy. Ultimately, microfluidic systems are envisioned to become a critical element of the development process for novel approaches to cancer treatment that will lead to significant improvements in patient outcomes.
Biography
Jeffrey T Borenstein, PhD, is Laboratory Technical Staff at Draper in Cambridge, Massachusetts. Dr Borenstein is a Principal Investigator for projects involving the application of microsystems technology towards organ assist devices and organ and disease models for drug efficacy and safety testing, as well as implantable drug delivery systems. Prior to joining Draper in 1994, Dr Borenstein held positions as a Research Scientist for North American Philips Corporation and Mobil Corporation. Dr Borenstein has a PhD in Physics and holds 47 issued patents and has written over 100 peer-reviewed journal articles and conference proceedings. He is also a member of the National Academy of Inventors and a Standing Member of the ISD panel for the National Institutes of Health.
References
- Mehlen P, Puisieux A. Metastasis: A question of life or death. Nature Reviews Cancer. 2006;6:449-58.
- Sackmann EK, Fulton AL, Beebe DJ. The present and future role of microfluidics in biomedical research. Nature. 2014;507:181-9.
- Bhatia SN, Ingber DE. Microfluidic organs-on-chips. Nature Biotechnol. 2014;32:760-2.
- Borenstein JT, Terai H, King KR, Weinberg EJ, Kaazempur-Mofrad MR, Vacanti JP. Microfabrication technology for vascularized tissue engineering. Biomed. Microdev. 2002;4:167-75.
- Zervantonakis IK, Hughes-Alford SK, Charest JL, Condeelis JS, Gertler FB, Kamm RD. Three-dimensional microfluidic model for tumor cell intravasation and endothelial barrier function. Proc. Nat. Acad. Sci. 2012;109:13515-20.
- Pardoll DM. The blockade of immune checkpoints in cancer immunotherapy. Nat. Rev. Cancer. 2012;12:252-64.
- Holton AB, Sinatra FL, Kreahling J, Conway AJ, Landis DA, Altiok S. Microfluidic Biopsy Trapping Device for the Real-Time Monitoring of Tumor Microenvironment. PLOS One. 2017;12:e0169797.
- Moore N, Doty D, Santos J, Kratchman L, Angione S, Mott V, Zielstorff M, Chen H, Gimbel A and Borenstein JT. A microfluidic model for real-time monitoring of tumor-lymphocyte interactions, AACR 2017. Late News Abstracts, Washington DC, April 4.
- Levine BL. Performance-enhancing drugs: design and production of redirected chimeric antigen receptor (CAR) T cells. Cancer Gene Ther. 2015;22:79-84.
- Fiering J. Acoustic separation for purification of lymphocytes. ISCT-NA Proceedings, Memphis TN, Sept 30, 2016.
Excellen paper