Manufacturing of CAR T-cell therapies: an overview
Posted: 18 February 2021 | Maciej Nakoniecznik (University College London) | No comments yet
The emergence of CAR T-cell therapies has ignited a revolution in the field of cancer treatments. While existing products show outstanding curative capability, they are hampered by significant clinical and commercial challenges, many of which stem from their manufacturing process. Maciej Nakoniecznik discusses how a combination of biological and technological advances in the field may allow CAR T cells to reach their full therapeutic potential.
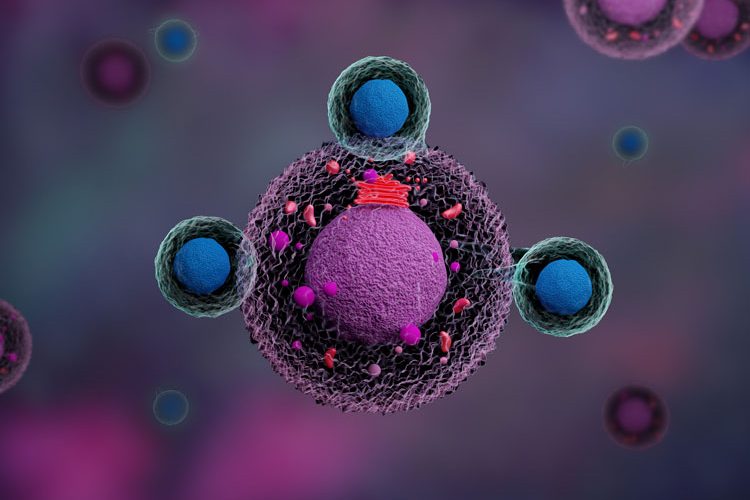
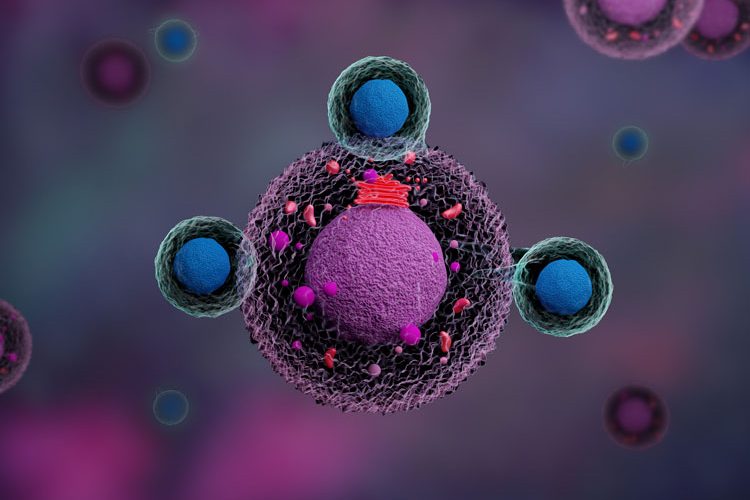
Chimeric antigen receptor (CAR) therapies target cancerous cells by utilising genetically modified T lymphocytes. This approach combines the specificity of a high-affinity, antibody-derived recognition domain with the cytolytic capabilities of T cells. Contrary to their natural mechanism of action, which involves the presentation of the antigen by the major histocompatibility complex (MHC) and the activation of the T-cell receptor (TCR), CAR T cells can recognise and destroy target cells directly.
First-generation CAR constructs comprised three distinct elements: the antigen-binding domain, the transmembrane domain connected to it by the hinge, and the intracellular CD3ζ signalling domain. The following CAR generations contain additional co-stimulatory domains that enhance their clinical efficacy. A range of co-stimulatory domains have been evaluated for use in CAR T cells and their effect on the cells varies from an increase in potency and cytolytic activity to improved in vivo persistence.1
Existing CAR T-cell products
Three CAR-T therapies have been approved by the US Food and Drug Administration (FDA) and the European Medicines Agency (EMA) as of January 2021. The most recent marketing authorisation was issued by EMA in December 2020.2
- Tisagenlecleucel (Kymriah; Novartis, Switzerland) was approved by the FDA in August 2017 for use in patients with relapsed or refractory diffuse large B-cell lymphoma or acute lymphoblastic leukaemia; EMA marketing authorisation followed a year later. Kymriah manufacturing takes place in facilities in France, Switzerland, Germany and the US and in October 2020 a new manufacturing plant in Kobe was approved by the Japanese regulators.3 Worldwide sales of the drug reached $278 million in 2019, up from $76 million the year before.4
- Axicabtagene ciloleucel (Yescarta; Kite Pharma/Gilead, US) received FDA approval in October 2017 for the treatment of several B-cell lymphomas. The EMA authorisation was issued in August 2018, together with Kymriah. Yescarta is manufactured in several facilities in the US and a production plant in the Netherlands capable of delivering 4,000 doses per year for European patients was approved in June 2020.5 Worldwide drug sales rose to $456 million in 2019.4
- Brexucabtagene autoleucel (Tecartus; Kite Pharma/Gilead, US) was issued an FDA approval in July 2020 for treatment of relapsed or refractory mantle cell lymphoma. EMA authorisation was granted in December 2020. The manufacturing currently takes place in Kite’s facility in El Segundo, California.6
All three products target the B lymphocyte CD19 antigen and their shared advantage is remarkable clinical efficacy. When used in paediatric and adult patients after multiple rounds of standard treatments, complete remission rates of 50‑80 percent were observed during clinical trials. These results are supported by post-approval analysis of Yescarta and Kymriah performance.7-9
A range of co-stimulatory domains have been evaluated for use in CAR T cells
Current CAR T-cell products clearly offer an outstanding treatment option for patients who were unresponsive to standard therapies; however, several obstacles are preventing them from having a wider impact on patient populations. Firstly, the approved products only target haematological malignancies, which form a minority of all new cancer cases diagnosed. The therapies are also associated with severe side effects such as cytokine release syndrome (CRS) and neurotoxicity, which have been observed in over 90 percent of patients in some trials.9,10 Finally, prices in the region of $373,000-$475,000 per treatment are a strong deterrent against their introduction in many healthcare systems.
The current CAR-T manufacturing process
CAR T-cell treatments currently available on the market are autologous in nature – ie, the patient is both the donor and the recipient of the cells. This forces manufacturers to scale out rather than scale up the process during commercialisation, which defines the nature of all processing steps.11-13
Leukapheresis: the first step usually takes place in a clinical setting. A patient’s peripheral blood mononuclear cells are collected, while the remainder of the blood is returned to the circulation. Initial T-cell enrichment may also be performed by counterflow centrifugal elutriation, a technique whereby cells are separated according to size or by density gradient centrifugation.
T-cell isolation and activation: Several antibody-based techniques for further T-cell purification have been developed. Fluorescence-activated cell sorting allows for isolation of specific subpopulations, such as CD4+ or CD8+ T cells, resulting in better control of product composition. However, low throughput and recovery make this method difficult to use in an industrial setting. Antibody-coated magnetic beads targeting the CD3 and CD28 surface antigens allow for simultaneous isolation and activation; however, this requires the addition of a bead removal step at the end of the process. Other T-cell activation methods include the use of anti-CD3 antibodies in the presence of IL-2 or the exposure of T cells to artificial antigen-presenting cells (aAPCs).
Genetic modification: following the enrichment steps, the CAR gene is introduced into the cells. Lentiviral and retroviral vectors are commonly used for this purpose, with the lentivirus generally showing higher gene transfer efficiency and expression stability. Viral gene transfer vectors require a separate manufacturing process with strict quality control. This leads to a major cost increase for the therapeutic cell manufacturer; therefore, alternative solutions are being actively explored. Transposon/transposase plasmid systems are a comparably inexpensive transfection method, but further research is needed regarding their efficacy and safety due to potential insertional mutagenesis. CRISPR-Cas9 holds great promise for a more controlled gene delivery method; however, its efficiency in introducing sufficiently large gene constructs is still being explored.
Cell expansion: the modified cells need to be expanded to the quantities of about 0.5-6.0×108 per patient. The process takes approximately 14‑21 days and is another major cost driver since cell culture practices used in research are often poorly translatable to industrial manufacturing. Traditional plate- and flask‑based cultures are labour intensive, introduce the possibility of operator error and require a cleanroom environment. Furthermore, serum- and animal‑free culture media must be used, which may lead to slower cell growth. Several novel expansion platforms that allow for automated, closed processing and in-line process monitoring have been gradually introduced in recent years. One of the most advanced examples is the Miltenyi CliniMACS Prodigy system, capable of performing automated cell isolation and expansion.
Formulation: at the end of the expansion process, the cell culture may reach volumes of up to five litres; the cell suspension must therefore be concentrated, usually to a volume of about 10-50mL. The cells are suspended in an infusible medium and cryopreserved for the duration of transportation to the clinic where they are thawed and administered to the patient.
The future of CAR T-cell therapies
Several challenges, both at the scientific and engineering level, must be addressed before CAR T-cell therapies for haematological cancers can reach their full potential. As mentioned earlier, severe treatment-associated toxicities limit their use in the clinic. The risk of CRS, caused by overactivation of CAR T cells, can potentially be decreased by including molecular “kill switches” in the CAR gene constructs, which would allow for rapid termination of the treatment. Elimination of on-target off-tumour toxicities (such as B-cell depletion) caused by CARs recognising the tumour-associated antigens (TAAs) on healthy cells would require modification of molecular targets for the therapies or changes in the cell activation mechanism.
Several challenges, both at the scientific and engineering level, must be addressed before CAR T-cell therapies for haematological cancers can reach their full potential
Targeting solid tumours presents another set of unique challenges. Immunosuppressive tumour microenvironment prevents T-cell migration to the site and hampers their activity by nutrient depletion and creation of acidic, hypoxic conditions. Clear molecular targets (such as CD19 in the case of B-cell malignancies) are scarce. Even if they can be identified, their frequent expression in healthy tissues of the body may lead to on-target off-tumour toxicity. However, with several novel approaches in development, such as CAR-T treatments in combination with checkpoint blockade therapies, there is hope for successful targeting of solid tumours in the future.
Scientific advances must be matched by improvements in manufacturing technologies if CARs are to make a widespread impact. Challenges associated with the autologous approach begin at the moment of cell collection, as their transport from the clinic to the manufacturing site presents issues with cell preservation and contamination. The cells are obtained from severely ill patients, often after multiple previous treatments. The starting quality of the cells will therefore vary greatly, impacting the rest of the manufacturing steps from cell expansion potential to transduction efficiency. Material availability for release testing is also a key issue, due to the length of time needed to reach the required therapeutic dose of the cells.
These key challenges could be largely resolved by moving from the autologous to the allogeneic therapeutic modality. Large-scale manufacturing of cells from a universal, healthy donor would, to an extent, resemble the bioprocesses known from the monoclonal antibodies industry. These are more closely related to traditional manufacturing, potentially decreasing overall costs. If the concerns around lower efficacy and immune rejection are resolved, allogeneic therapies may become the future of the sector.
As a final remark, it may be beneficial to consider a new, systemic approach to CAR-T therapies as a whole, especially in the current absence of approved allogeneic products. CAR T-cell therapies can be considered more as a service than a pharmaceutical product. Each manufacturing process is different, depending on the starting condition of the patient, their location in relation to the production site and the possible therapy administration times. The success of the treatment also depends on the co-operation of multidisciplinary teams of manufacturers and clinicians and, finally, CAR T cells promise to deliver an effective cure within a single dose. Awareness of these key differences between CAR T cells and traditional therapeutic approaches is critical in order for healthcare systems and policy makers to appreciate their full significance and to facilitate their expansion into the market.
About the author
Maciej Nakoniecznik
Maciej graduated with a BSc in Bioprocessing of New Medicines from University College London, receiving numerous academic awards. He is currently pursuing an MSc in Manufacture and Commercialisation of Stem Cell and Gene Therapies at the same institution. He has worked on a range of academic projects involving bioprocess design, centrifugation modelling and genomic data analysis, where he is gaining substantial research experience.
References
1. Labanieh L, Majzner R, Mackall C. Programming CAR-T cells to kill cancer. Nature Biomedical Engineering. 2018;2(6):377-391.
2. Committee for Medicinal Products for Human Use (CHMP). CHMP summary of positive opinion for Tecartus. European Medicines Agency; 2020 p. 1-2.
3. Blankenship K. Novartis fills manufacturing gap for CAR-T therapy Kymriah with first Asian production facility. Fierce Pharma. 2020.
4. Huang J. Affordability issues to cloud upcoming debut of cancer cell therapy in China. S&P Global Market Intelligence. 2020.
5. Hargreaves B. Kite able to manufacture Yescarta in Europe after approval. BioPharma Reporter. 2020.
6. Gilead. U.S. FDA Approves Kite’s Tecartus™, the First and Only CAR T Treatment for Relapsed or Refractory Mantle Cell Lymphoma. 2020.
7. Master A. Kymriah vs. Yescarta. Nucleus Biologics; 2019.
8. Food and Drug Administration. FDA Approves First Cell-Based Gene Therapy For Adult Patients with Relapsed or Refractory MCL. 2020.
9. Elsallab M, Levine B, Wayne A, Abou-El-Enein M. CAR T-cell product performance in haematological malignancies before and after marketing authorisation. The Lancet Oncology. 2020;21(2):e104-e116.
10. Santomasso B, Bachier C, Westin J, Rezvani K, Shpall E. The Other Side of CAR T-Cell Therapy: Cytokine Release Syndrome, Neurologic Toxicity, and Financial Burden. American Society of Clinical Oncology Educational Book. 2019;(39):433-444.
11. Levine B, Miskin J, Wonnacott K, Keir C. Global Manufacturing of CAR T Cell Therapy. Molecular Therapy: Methods & Clinical Development. 2017;4:92-101.
12. Iyer R, Bowles P, Kim H, Dulgar-Tulloch A. Industrializing Autologous Adoptive Immunotherapies: Manufacturing Advances and Challenges. Frontiers in Medicine. 2018;5.
13. Wang X, Rivière I. Clinical manufacturing of CAR T cells: foundation of a promising therapy. Molecular Therapy – Oncolytics. 2016;3:16015.
Issue
Related topics
Anti-Cancer Therapeutics, Biopharmaceuticals, Bioprocessing, Bioproduction, t-cells, Therapeutics
Related organisations
European Medicines Agency (EMA), University College London (UCL), US Food and Drug Administration (FDA)