Separation and purification applications for mutagenic impurities
Posted: 29 June 2017 | Dave Elder (JPAG Member and David P Elder Consultancy) | No comments yet
Classically, isolation of key intermediates and the resultant active pharmaceutical ingredient (API) has formed the basis of clean up and purification strategies within the pharmaceutical industry…
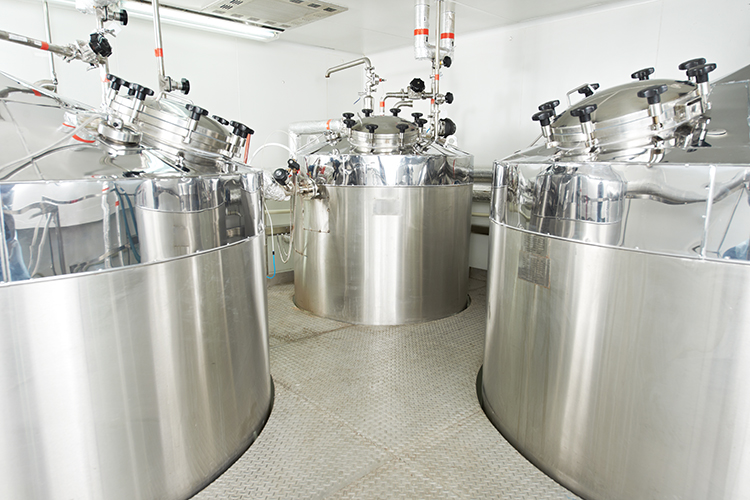
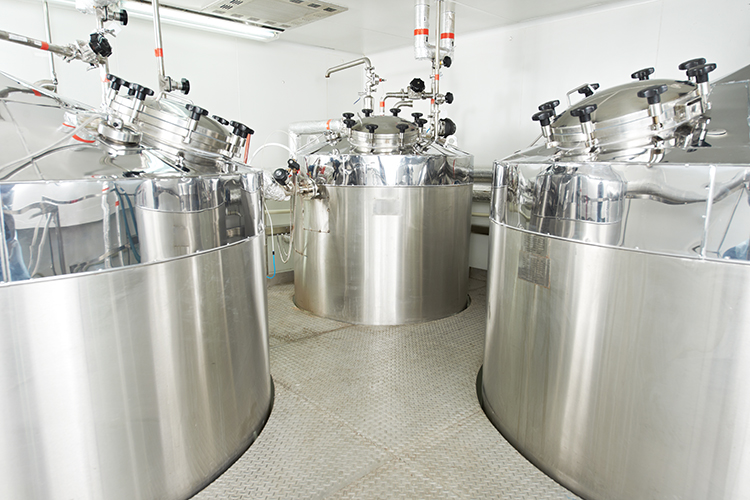
Crystallisation, and sometimes recrystallisation, are recognised as the main separation and purification processes during the manufacture of APIs.1 The advent of guidance for mutagenic impurities (MI) and potentially mutagenic impurities (PMIs)2 has necessitated control of these very toxic impurities at very low levels (often ppm) in APIs. Although the purging capability of many synthetic processes are typically very good;3 in some cases purging, allied with classical crystallisation/isolation, cannot offer sufficient control and supplementary purification strategies are required.
Preparative high performance liquid chromatography (prep-HPLC) has become a standard technique for the removal or reduction of impurities from APIs and it is available at multi-kg scale, both at contract facilities and embedded within large pharmaceutical companies. It provides numerous advantages, such as high efficiency and good reproducibility of the separation. Prep-HPLC is typically carried out in normal-phase mode;3 the driving force is the necessity for rapid removal of the solvent and isolation of the intermediate/API.4
Maddula et al5 described the development of a preparative HILIC (hydrophilic interaction chromatography) approach for the removal of a structurally related mutagenic dimer impurity from rizatriptan API. The approach used a combination of molecular structural descriptors, together with related physicochemical descriptors, eg, pKa, LogP and LogD. In addition, the optimal selection of ionic strength and mobile phase pH can accelerate resolution and enhance the robustness of the methodology.
Counter-current chromatography (CCC)
Counter-current chromatography (CCC), in contrast to the more common prep-HPLC, is more tolerant of particulate matter, shows good reproducibility and utilises less expensive stationary phases.6,7 Although CCC was first developed in the early 1960s, it wasn’t until the early part of this century that the technique was commercialised and, more importantly, scaled up to allow separations on the kg scale. Sutherland et al6 reported on the purification of a crude mixture (87% pure) of a novel API, to achieve a target purity of >95% with the ‘total absence’ of a PMI, the bromo-analogue of the API. High performance CCC was utilised with a silica column using a non-polar mobile phase with a flow rate of 1mL/minute and a rotational speed of 2,100rpm. The sample loading was 200mg. The run time was 50 minutes, with the bromo-impurity eluting between 15-25 minutes and the API eluting after this. The API recovery was 59%.6
The main challenges inherent in adapting this new technology is the lack of experience with CCC compared to the existing prep-HPLC. The other challenges include high API losses,^6 reliability of column performance (reproducibility/scalability), costs, applicability to purifying ‘very dirty’ materials and to compounds that are intrinsically unstable on silica columns, waste handling, capability, speed, and ease of use.
Supercritical fluid chromatography (SFC)
Preparative supercritical fluid chromatography (SFC) is widely used in discovery to purify intermediates. The benefits include different selectivity, enhanced purity, lower costs and better environmental footprint. Biba and Liu8 described purification of an aromatic amine PMI using both SFC and the more established ‘flash’ liquid chromatography (Table 1). It can be readily seen that SFC showed superior performance to the classical approach.
Parameter | ‘Flash’ Chromatography | SFC |
Purity | Failed to purify sample | >99% |
Processing Rate (g/min) | 0.025 | 0.027 |
Separation Time (mins) | 40 | 110 |
Solvent Usage (L) / Analyte (g) (g/L) | 1.6 | 0.76 |
Scale (g) | 1 | 3 |
Table 1: Comparison of ‘flash’ chromatography versus preparative SFC for the purification of a discovery intermediate (adapted from Biba and Liu, 2016)8
Organic solvent nanofiltration (OSN)
Organic solvent nanofiltration (OSN) utilises medium pressure separation of the lower molecular weight MIs from the higher molecular weight APIs. This is typically ≥20bar, but this is dependent on the polymeric membrane used. The MIs are removed from the system by filtration, whereas the API/intermediate are retained on the polymeric membrane (ca 5µM). The process is applicable to molecules up to 1,000 Daltons. OSN can be operated in a diafiltration mode, ie, in a steady state, where solvent is continuously added to compensate for losses to the membrane.9 Székely et al9 used 11 model MIs, representing several different chemical classes, ie, ureas, aldehydes, amides and sulfonate esters, with molecular weights varying from 56 to 224 Daltons, with an average of 130 Daltons and nine different model APIs, with molecular weights varying from 170 to 837 Daltons, with an average of 415 Daltons.
The optimal solvents based on API solubility were methyl ethyl ketone (MEK) and tetrahydrofuran (THF). Conversely, the superior solvating powers of these solvents made it challenging to select appropriate OSN commercially available polymeric membranes.
However, the authors did indicate that the methodology is generic, scalable, and can be applied to other APIs, MIs/PMIs, solvents and membranes. The general recommendation is to select solvents/polymeric membranes that give a high rejection value for the designated APIs, ie, >95%, and to extend the number of ‘diavolumes’ aligned with optimal MI removal efficiencies. Three case studies were reported9 and in all cases API rejection rates were high. For low dose drugs, ie, 5mg/day, where the allowable MI limit based on TTC values2 is commensurately higher, ie, 300 ppm, the number of ‘diavolumes’ used were lower (two) and the API losses were generally lower (1.8%). In contrast, for higher dose drugs (≥150mg/day), where the allowable MI limit is much lower,2 (10ppm), then the number of ‘diavolumes’ required is higher, (seven) and the API losses are commensurately higher (5.5%).
Insufficient discrimination between the API and the MI, together with the reactive nature of the MI causing deterioration in membrane performance,11,12 limits the industrial applications of OSN. In addition, OSN can be used in the counter-current cascade mode to improve separation efficiencies.11 Kim et al13 implemented a two-stage cascade configuration and the process yield was increased from 58% to 95%, while maintaining MI levels of less than 5ppm of 4-dimethylaminopyridine (DMAP) and ethyl tosylate (EtTS) in the final solution. Peeva et al14 demonstrated the continuous purification of an API from an MI using a two-stage membrane cascade. The API purity could be increased from 78% to greater than 99%. Bromoethane could be successfully removed from an API despite the small difference in molecular weight, ie, only 33 Daltons.15
OSN fractionation was also recently performed using a three-stage cascade.16,17 Purification of the initial feed solution, containing an API and an excess reagent, produced an API-rich product stream. This API-rich stream could be further purified by crystallisation, which was impossible with the original feed solution.17 OSN can sometimes be used in the ‘reverse’ mode, whereby the API, due to its small size, passes through the membrane and the MI is retained. Pink et al18 retained the PMI on the polymeric membrane, whilst the API could be additionally purified after passing through the membrane.
Molecular imprinted polymers (MIPs)
Molecular imprinted polymers (MIPs) are well characterised, polymeric materials designed to recognise specific analytes (MIs/PMIs) with very high affinities. During the polymerisation process the template (ie, the proposed MI/PMI) forms a stable complex with the monomer in the presence of a cross-linker and ‘porogen’, which effectively constrains the MIP within a rigid, porous, polymeric matrix system, ie, a ‘lock-and-key’ system.19 Synthetic MIPS have very high affinities, with low cost and are stable at high pressures and temperatures, and in the presence of acidic, basic and organic systems. MIPs have been used for the removal of IPU,10 acetamide,20 arylsulfonates,21 acrolein,22 ureas,23 and aminopyridines.24
Székely et al10 assessed the removal of IPU from MF using MIP technologies. They demonstrated high removal of IPU at levels ≤ 100ppm in a single stage process (83%). The selectivity and stability of the MIP polymer was validated by repetitive scavenging (x18) of the MI using the same absorbent. They did see non-specific binding of the API to some MIPs, but by using a combination of OSN/MIP they could address this issue. This resulted in 50-fold reduction in the levels of IPU in MF.
Székely et al20 evaluated the scavenging of acetamide from another steroid API, halobetasol propionate. The MIP showed very good selectivity for acetamide in the presence of other short chain alkyl amides, eg, formamide, acrylamide, N–tert-butylacrylamide, or aryl amides, eg, benzamide. In addition to good selectivity for the MI, the MIP showed very low binding towards the API.
Esteves et al^25 assessed the scavenging ability of MIPs towards 4-DMAP in MF. The polymers effectively removed the MI 4-DMAP at levels of 256ppm in the API (88% removal), with high API recoveries (>96%). By combining OSN with MIP, quantitative removal of the MI (>99%) can be achieved with high recoveries of the API (>92%). Recent applications of L-phenylalanine methyl ester MIPS, showed the most robust behaviour. These polymers can be reused at least 100 times without loss of affinity towards the MI-templated analyte under acidic and basic conditions, at elevated temperature (65°C). In contrast, the performance of acrylamide-based or methacrylate-based polymers does deteriorate over time under both acidic and basic extraction conditions.26
However, there are some green chemistry’ disadvantages for conventional MIPs, such as the use of large volumes of organic solvents and the need to increase the specific surface area (SSA) using milling techniques.27 In contrast, supercritical fluid (SCF) can be used as an alternative, ‘green’ solvent to produce MIPs as free-flowing powders with high SSA, in very high yields. Viverios et al27 used acetamide as their model MI template, a methacrylate polymer using methacrylic acid and methacrylamide as the monomers and ethylene glycol dimethacrylate as the cross-linker. They demonstrated very high capacity (up to 2.5-fold reduction) and high affinity towards acetamide at 250ppm levels, in comparison to other low molecular weight amides, eg, benzamide and pivalamide.
Nucleophilic reactive resins
In contrast to separation or adsorption approaches, the removal mechanism for nucleophilic reactive resins is based on a selective reaction between the electrophilic MI and a nucleophilic site on the resin. Lee et al28 screened several nucleophilic resins that all included an amino or thiol moiety, and the optimal approach used an ethylene diamine or trimercaptotriazine resin, respectively. Solutions of sulfonate ester MIs (100 ppm) in various organic solvents in the presence of selected APIs were treated with nucleophilic resins at a high resin load. The API does not interfere with the removal of the electrophiles and API losses were minimal (<10%). The removal efficiency is inversely proportional to the alkyl chain length of the sulfonate ester (methyl sulfonate esters (100%) > ethyl sulfonate esters (30%) > iso-propyl sulfonate esters (20%)). Other electrophilic MIs, ie, alkyl and aryl halides, can also be removed. However, the stability of these polymeric resins in the presence of THF has been contraindicated. This solvent can leach monomers from the resin and this has largely precluded the use of these resins in large-scale synthetic processes.4
Conclusion
Although a number of alternative separation approaches have been applied to difficult purification issues, the reality is that these approaches will never attain widespread utility at commercial scales. This is principally because of the complexity, high API losses, and adverse effect on the API cost of goods. However, these approaches can be still gainfully applied in discovery and early-development, where time lines are the most important parameter and cost is less of an issue.
Biography
References
- Fujiwara M, Nagy ZK, Chew JW, Braatz RD. First-principles and direct design approaches for the control of pharmaceutical crystallization. J. Proc. Control. 2005;15:493-504.
- ICH M7, 2014. Assessment and control of DNA reactive (mutagenic) impurities in pharmaceuticals to limit potential carcinogenic risk. Current Step 4 version. 23 June 2014.
- Teasdale A, Elder DP, Chang S-J, Wang S, Thompson R, Benz N, Sanchez Flores IH. Risk Assessment of Genotoxic Impurities in New Chemical Entities: Strategies to Demonstrate Control. Proc. Res. Dev. 2013;17:221-230.
- Teasdale A, Elder DP, Fenner S. Strategies for the evaluation of genotoxic risk. In: Genotoxic impurities: Strategies for Identification and Control, Ed. Teasdale A. John Wiley and Sons, Inc. 2010. pp.221-248.
- Maddula SR,Kharkar M, Manudhane K, Kale S, Bhori A, Lali A, Dubey PK, Sarma KRJ, Bhattacharya A, Bandichhor Preparative Chromatography Technique in the Removal of Isostructural Genotoxic Impurity in Rizatriptan: Use of Physicochemical Descriptors of Solute and Adsorbent. Org. Proc. Res. Dev. 2009;13(4):683-689.
- Sutherland I, Ignatova S, Hewitson P, Janaway L, Wood P, Edwards N, Harris G, Guzlek H, Keay D, Freebairn K, Johns D, Douillet N, Thickitt C, Vilminot E, Matthews B. Scalable technology for the extraction of pharmaceutics (STEP): The transition from academic knowhow to industrial reality. J. Chromatographr. A. 2011;1218:6114-6121.
- Sutherland I, Thickitt C, Douillet N, Freebairn K, Johns D, Mountain C, Wood P, Edwards N, Rooke D, Harris G, Keay D, Matthews B, Brown R, Garrard I, Hewitson P, Ignatova S. Scalable technology for the extraction of pharmaceutics (STEP): Outcomes from a 3 year collaborative industry/academia research programme. J. Chromatographr. A. 2013;1282:84-94.
- Biba M, Liu J. 2016. A perspective on the application of preparative supercritical fluid chromatography using achiral stationary phases in pharmaceutical drug discovery and development. Amer. Pharm. Review. April 30.2016; (1-7). http://www.americanpharmaceuticalreview.com/Featured-Articles/185891-A-Perspective-on-the-Application-of-Preparative-Supercritical-Fluid-Chromatography-Using-Achiral-Stationary-Phases-in-Pharmaceutical-Drug-Discovery-and-Development/. Accessed on 16 May 2017.
- Székely G, Bandarra J, Heggie W, Sellergreen B, Ferreira FC. 2011. Organic solvent nanofiltration. A platform for removal of genotoxins from active pharmaceutical ingredients. J. Memb. Sci. 2001:381:21-33.
- Székely G, Bandarra J, Heggie W, Sellergreen B, Ferreira FC. 2012. A hybrid approach to reach stringent low genotoxicity impurity contents in active pharmaceutical ingredients: Combining molecularly imprinted polymers and organic solvent nanofiltration for removal of 1,3-diisopropyurea. Sep. Purif. Technol. 2012;86:79-87.
- Marchetti P, Jimenez Solomon MF, Szekely G, Livingston AG. Molecular Separation with Organic Solvent Nanofiltration: A Critical Review. Rev. 2014;114: 10735-10806.
- Bandarra J, Heggie W, Szekely, G. 2012. Organic solvent nanofiltration for the removal of genotoxic impurities from APIs. PT105601(A).
- Kim JF, Szekely G, Valtcheva IB, Livingston AG. Increasing the sustainability of membrane processes through cascade approach and solvent recovery—pharmaceutical purification case study. Green Chem. 2014;16:133.
- Peeva L, da Silva Burgal J, Valtcheva I, Livingston AG. Continuous purification of active pharmaceutical ingredients using multistage organic solvent nanofiltration membrane cascade. Chem. Eng. Sci. 2014;116:183.
- Vanneste J, Ormerod D, Theys G, Van Gool D, Van Camp B, Darvishmanesh S, Van der Bruggen B. Towards high resolution membrane-based pharmaceutical separations. J. Chem. Technol. Biotechnol. 2013;88:98-108.
- Siew W, Livingston AG, Ates C, Merschaert A. Molecular separation with an organic solvent nanofiltration cascade – augmenting membrane selectivity with process engineering. Chem. Eng. Sci. 2013;90:299-310.
- Siew WE, Livingston AG, Ates C, Merschaert A. Continuous solute fractionation with membrane cascades – A high productivity alternative to diafiltration. Sep. Purif. Technol. 2013;102:1-14.
- Pink CJ, Wong H, Ferreira FC, Livingston AG. Organic Solvent Nanofiltration and Adsorbents; A Hybrid Approach to Achieve Ultra Low Palladium Contamination of Post Coupling Reaction Products. Org. Process Res. Dev. 2008;12:589-595.
- Whitcombe MJ, Kirsch N, Nicholls LA. Molecular imprinting science and technology: A survey of the literature for the years 2004-2011. J. Molec. Recogn. 2014;27:297-401.
- Székely G, Fritz E, Bandarra J, Heggie W, Sellergreen B. Removal of potentially genotoxic acetamide and arylsulfonate impurities from crude drugs by molecular imprinting. J. Chromatographr. A. 2012;1240:52-58.
- Kecili R, Billing J, Leeman M, Nivhede D, Sellergren B, Rees A, Yilmaz E. Selective scavenging of the genotoxic impurity methyl p-toluenesulfonate from pharmaceutical formulations. Sep. Purif. Technol. 2013;103:173.
- Kecili R, Nivhede D, Billing J, Leeman M, Sellergren B. Yilmaz E. Removal of Acrolein from Active Pharmaceutical Ingredients Using Aldehyde Scavengers. Process Res. Dev. 2012;16:1225.
- Székely G, Bandarra J, Heggie W, Ferreira FC, Sellergreen B. Design, preparation and characterization of novel molecularly imprinted polymers for removal of potentially genotoxic 1,3-diisopropyurea. Sep. Purif. Technol. 2012;86:190-198.
- Kecili R, Billing J, Nivhede D, Sellergren B, Rees A, Yilmaz E. J. Fast identification of selective resins for removal of genotoxic aminopyridine impurities via screening of molecularly imprinted polymer libraries. J. Chromatogr. A. 2014;1339:65.
- Esteves T, Viveiros R, Bandarra J, Heggie W, Casimiro T, Ferreira FC. Molecular imprinted polymer strategies for removal of a genotoxic impurity, 4-dimethylaminopyridine, from an active pharmaceutical ingredient post-reaction stream. Sep. Purif. Technol. 2016;163:206-214.
- Kupai J, Razali M, Buyuktiryaki S, Kecilic R, Szekely G. Long-term stability and reusability of molecularly imprinted polymers. Polym. Chem. 2017;8:666-673.
- Viveiros R, Lopes MI, Heggie W, Casimiro T. Green approach on the development of lock-and-key polymers for API purification. Chem. Eng. J. 2017;308:229-239.
- Lee C, Helmy R, Strulson C, Plewa J, Kolodziej E, Antonucci V, Mao B, Welch CJ, Ge Z, Al-Sayah MA. Removal of Electrophilic Potential Genotoxic Impurities Using Nucleophilic Reactive Resins. Org. Proc. Res. Dev. 2010;14(4):1021-1026
To view references, please visit: www.europeanpharmaceuticalreview.com/3/-17-Elder
Issue
Related topics
Active Pharmaceutical Ingredient (API), Chromatography, HPLC, Manufacturing